SUMMARY
Each day the adult human produces approximately 1 × 1011 platelets, a level of production that can increase 10- to 20-fold in times of increased demand and an additional five- to 10-fold under the stimulation of exogenous thrombopoietin mimetic drugs. Production of platelets depends on the proliferation and differentiation of hematopoietic stem and progenitor cells to cells committed to the megakaryocyte lineage, their maturation to large, polyploid megakaryocytes, and their final fragmentation into platelets. The external influences that impact megakaryopoiesis and thrombopoiesis are a supportive marrow stroma consisting of endothelial and other cells, matrix glycosaminoglycans, and a family of protein hormones and cytokines, including thrombopoietin, stem cell factor, and stromal cell-derived factor-1. The role of the cytokines essential for these processes has been defined, the transcription factors critical for megakaryocyte development have been identified, the molecular mechanisms that underlie the two most unusual aspects of thrombopoiesis—endomitosis and proplatelet formation—have been studied, and reagents to specifically modify platelet production have been generated. This chapter focuses on the development of megakaryocytes, their precursors and their progeny, and the hematopoietic growth factors and transcriptionally active molecules that control the survival, proliferation, and differentiation of these cells.
The circulatory life span of a platelet is approximately 10 days in humans with normal platelet counts, but somewhat shorter in patients with moderate (7 days) to severe (5 days) thrombocytopenia, as a higher proportion of the total-body platelet mass is consumed in the day-to-day function of maintaining vascular integrity.1 Based on a “normal” level of 200,000 platelets/μL, a blood volume of 5 L, and a half-life of 10 days, 1 × 1011 platelets per day are produced. If 1 megakaryocyte produces approximately 1000 platelets, approximately 1 × 108 megakaryocytes are generated in the marrow each day.
Acronyms and Abbreviations:
CAMT, congenital amegakaryocytic thrombocytopenia; FGF, fibroblast growth factor; GP, glycoprotein; HPS, Hermansky-Pudlak syndrome; IFN, interferon; IL, interleukin; ITP, immune thrombocytopenic purpura; MAPK, mitogen-activated protein kinase; P4P, polyphosphate-4-phosphatase; PI3K, phosphoinositol 3′-kinase; SDF, stromal cell-derived factor; TGF, transforming growth factor.
Several independent lines of evidence indicate the transit time from megakaryocyte progenitor cell to release of platelets into the circulation ranges from 4 to 7 days. For example, following platelet apheresis, the platelet count falls, recovers substantially by day 4, and completely recovers by day 7.2 In most physiologic and pathologic states, the platelet count is inversely related to plasma thrombopoietin levels. For example, liver failure is associated with moderate thrombocytopenia as a result of splenomegaly and thrombopoietin deficiency. Within the first week following orthotopic liver transplantation, the platelet count rises substantially, with kinetics matching those of thrombopoietin infusion.3,4 These findings indicate expansion of the megakaryocyte mass takes from 3 to 4 days following a thrombopoietin stimulus in humans and, coupled with the approximate 12 hours required for platelet release,5 results in a relatively brisk response to thrombocytopenia.
Platelets form by fragmentation of megakaryocyte membrane extensions termed proplatelets, in a process that consumes nearly the entire cytoplasmic complement of membranes, organelles, granules, and soluble macromolecules. Although at first controversial, as the process was initially observed only in vitro, in situ microscopic studies have identified proplatelet formation and fragmentation in living animals.6 Each megakaryocyte is estimated to give rise to 1000 to 3000 platelets7 before the residual nuclear material is engulfed and eliminated by marrow macrophages. This process has been extensively reviewed.8 The continuum of megakaryocyte development is arbitrarily divided into four stages. The major criteria differentiating these stages are the quality of the cytoplasm and the size, lobulation, and chromatin pattern of the nucleus (Table 1–1).
Term | Size (μM) | Morphology |
---|---|---|
Megakaryoblast (stage I) | >10 | Lobed nucleus, basophilic cytoplasm |
Basophilic megakaryocyte (stage II) | >20 | Horseshoe-shaped nucleus, basophilic cytoplasm, azurophilic granules around centrosome |
Granular megakaryocyte (stage III) | >25–50 | Large multilobed nucleus, acidophilic cytoplasm, numerous azurophilic granules |
Mature megakaryocyte (stage IV) | >25–50 | Pyknotic nucleus, groups of 10–12 azurophilic granules |
Stage I megakaryocytes, also termed megakaryoblasts, account for approximately 20 percent of all cells destined to form platelets. These cells in human marrow are 8 to 24 μm in spherical diameter (i.e., the actual size in vivo, as opposed to the apparent size of a cell on a flattened marrow smear) and contain a relatively large, minimally indented nucleus with loosely organized chromatin and multiple nucleoli, and scant basophilic cytoplasm containing a small Golgi complex, a few mitochondria and α granules, and abundant free ribosomes (Fig. 1–1).
Figure 1–1.
Electron micrograph of a normal human megakaryoblast stained for platelet peroxidase. The small cell (<9 μm) exhibits dense platelet peroxidase in the perinuclear space and endoplasmic reticulum (arrows) (magnification ×12,150). (Inset) Enlargement of the Golgi zone. The Golgi saccules and vesicles are devoid of platelet peroxidase (open arrows), whereas the endoplasmic reticulum contains platelet peroxidase activity (closed arrow) (magnification ×25,000). (Used with permission of Dr. J. Breton-Gorius.)
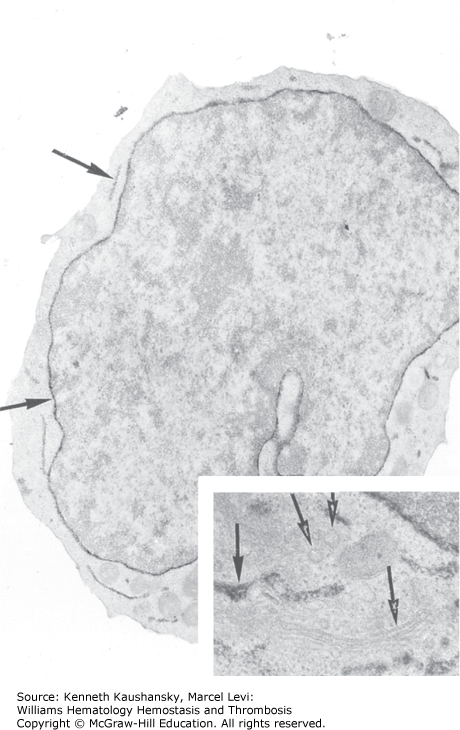
Although elegant experiments clearly demonstrated that the gene for integrin αIIb is expressed as early as the erythroid-megakaryocytic progenitor stage9 and possibly in the common myeloid progenitor, the cell-surface protein becomes demonstrable and functionally important only at the early stages of megakaryocyte development. Integrin αIIbβ3 is an integral transmembrane protein of two subunits, but only the α subunit is megakaryocyte-lineage specific. Absence of integrin αIIbβ3 leads to Glanzmann thrombasthenia resulting from failure of the defective platelets to engage fibrinogen and other adhesive ligands during hemostasis (Chap. 10). Megakaryocytes and platelets contain in their cytoplasmic membranes approximately twice the amount of integrin αIIbβ3 as is present on the cell surface. The granule compartment serves as a mobilizable pool that is exteriorized upon platelet activation. During the early and mid stages of megakaryocyte development, the granule content of integrin rises. Moreover, because developing megakaryocytes do not synthesize but contain fibrinogen in their α-granules and cells from patients with Glanzmann thrombasthenia do not, integrin αIIbβ3 clearly begins to function, at least at the level of fibrinogen binding and uptake, long before platelet formation.
The glycoprotein (GP) Ib-IX complex is expressed only slightly after the appearance of integrin αIIbβ3.10 Although endothelial cells reportedly express GPIb,11 its levels are very low; otherwise, GPIb is the second most abundant megakaryocyte-specific protein. Glycoprotein V also is expressed in complex with GPIb and GPIX, in a ratio of 1:2:2.12 However, the genetic elimination of GPV has little effect on platelet adhesion,13 and unlike GPIb and GPIX, no mutations of GPV are associated with Bernard-Soulier disease (Chap. 10).14 Therefore, GPV does not appear to be required for the GPIb-V-IX complex to function as a von Willebrand factor receptor. Rather, GPV is a target of thrombin, potentially playing a role in platelet activation.15
Another feature of the megakaryoblast is the initial development of demarcation membranes, which begin as invaginations of the plasma membrane and ultimately develop into a highly branched interconnected system of channels that course through the cytoplasm. The demarcation membrane system is in open communication with the extracellular space, based on studies using electron dense tracers.16 Biochemical analysis indicates the composition of these membranes is very similar to the plasma membrane at each stage of megakaryocyte development. Over the 72 hours required for stage III/IV cells to develop from megakaryoblasts, the demarcation membrane system grows substantially. The demarcation membrane system provides the material necessary for development of proplatelet processes, structures that form in stage IV megakaryocytes and give rise upon fragmentation to mature platelets.8,17
One of the most characteristic features of megakaryocyte development is endomitosis, a unique form of mitosis in which the DNA is repeatedly replicated in the absence of nuclear or cytoplasmic division. The resultant cells are highly polyploid. Endomitosis begins in megakaryoblasts (Fig. 1–2) following the many standard cell divisions required to expand the number of megakaryocytic precursor cells and is completed by the end of stage II megakaryocyte development.18 During the endomitotic phase, each cycle of DNA synthesis produces an exact doubling of all the chromosomes, resulting in cells containing DNA content from eight to 128 times the normal chromosomal complement in a single, highly lobated nucleus. Although poorly understood for many years, the ability to produce large numbers of normal megakaryocytes in culture has started to shed light on this enigmatic process. Endomitosis is not simply the absence of mitosis but rather consists of recurrent cycles of aborted mitoses.19 Cell-cycle kinetics in endomitotic cells also are unusual, characterized by a short G1 phase, a relatively normal DNA synthesis phase, a short G2 phase, and a very short endomitosis phase.20 During the endomitosis phase, megakaryocytic chromosomes condense, the nuclear membrane breaks down, and multiple (at advanced stages) mitotic spindles form upon which the replicated chromosomes assemble. However, following initial chromosomal separation, individual chromosomes fail to complete their normal migration to opposite poles of the cell, the spindle dissociates, the nuclear membrane reforms around the entire chromosomal complement, and the cell again enters G1 phase.
Figure 1–2.
Origin and development of megakaryocytes. The pluripotential stem cell produces a progenitor committed to megakaryocyte differentiation (colony-forming unit–megakaryocyte [CFU-MK]), which can undergo mitosis. Eventually the CFU-MK stops mitosis and enters endomitosis. During endomitosis, neither cytoplasm nor nucleus divides, but DNA replication proceeds and gives rise to immature polyploid progenitors, which then enlarge and mature into morphologically identifiable, mature megakaryocytes that shed platelets. This figure does not necessarily imply that endomitosis and platelet formation are sequential but they can occur simultaneously. Meg-CFC, megakaryocyte colony-forming cells.
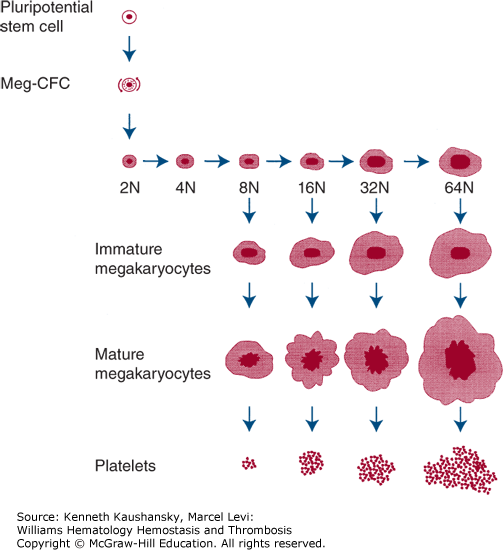
The promoters for integrin αIIb, GPIb, GPVI, GPIX, and platelet factor-4 genes have been the focus of several studies and are active at the megakaryoblast stage of development. Consensus sequences for both GATA-1 and members of the Ets family of transcription factors (e.g., Fli-1) are present in the 5′ flanking regions of these genes, deletion of which reduces or eliminates reporter gene expression,21–24 at least in mature hematopoietic cells. MafB also enhances GATA-1 and Ets activity during megakaryoblast differentiation,25 induced by activation of ERK1/2, one of the primary downstream events of thrombopoietin stimulation.26
Another target of GATA-1 in megakaryocytes is polyphosphate-4-phosphatase (P4P), which was first identified by subtraction cloning between normal and GATA-1 knockdown megakaryocytes.27 One of the unexplained features of megakaryocytes in GATA-1 knockdown mice is that, rather than massive cell death as seen in GATA-1–deficient erythroid progenitors,28 the aberrantly developing megakaryoblasts in GATA-1 knockdown marrow are highly abundant and proliferate in vitro far more than control cells.29 P4P catalyzes hydrolysis of the D-4 position phosphate of PI3,4P and PI3,4,5P. These membrane phospholipids are products of phosphoinositol 3′-kinase (PI3K) action on membrane phospholipids, and they play an important role in the proliferative and survival response to megakaryocyte growth factors. When reintroduced into the knockdown mice, P4P diminishes the exuberant growth characteristic of the knockdown cells.27 These findings are similar to the phenotype of cells from PTEN or SHIP knockout mice, enzymes that hydrolyze the D-3 and D-5 positions of PI3,4,5P.
Another transcription factor vital for megakaryoblast differentiation is RUNX1 (also termed CBFA2 and AML1), the gene responsible for thrombocytopenia seen in familial platelet disorder/predisposition to acute myelogenous leukemia (Chap. 9).30 In this disorder, haploinsufficiency of RUNX1 is associated with thrombocytopenia. As its genetic elimination in mice leads to significant maturation defects in the megakaryocyte lineage,31 the human disorder almost certainly results from this genetic alteration. During normal megakaryoblast differentiation, RUNX1 levels rise and, conversely, fall during erythroid differentiation. In response to phosphorylation by ERK1/2, RUNX1, in complex with CBFβ and together with GATA-1, induces integrin αIIb and integrin α2 expression in megakaryoblast-like cells,32 providing the beginnings of a molecular explanation for megakaryocyte development.
The cytokines, hormones, and chemokines that affect the survival and proliferation of megakaryoblasts include thrombopoietin, interleukin (IL)-3, stem cell factor (also termed mast cell growth factor, steel factor, and c-kit ligand), and the chemokine CXCL12 (previously termed stromal cell-derived factor [SDF]-1). Thrombopoietin is the most critical (for additional details, see the more extensive discussion in “Hormones and Cytokines” below), as genetic elimination of the TPO gene in mice leads to circulating platelet levels approximately 10 percent of normal. Homozygous or complex heterozygous mutation of the gene encoding the thrombopoietin receptor cMPL leads to congenital amegakaryocytic thrombocytopenia, in which platelet levels are approximately 10 percent of normal because of a near absence of megakaryocytic progenitors and megakaryoblasts (Chap. 7). The importance of stem cell factor to megakaryoblast development is revealed by experimental findings both in vitro and in vivo. Genetic reduction in expression of stem cell factor or its receptor c-kit leads to a 50 percent reduction in circulating platelet levels.33 The cytokine acts in synergy with thrombopoietin to enhance megakaryocyte production in semisolid and suspension culture systems.34 Evidence that IL-3 contributes to normal or accelerated megakaryopoiesis in vivo is weak. Genetic elimination of the IL-3 gene fails to affect platelet counts, even when combined with thrombopoietin receptor deficiency,35 but the cytokine can induce growth of marrow progenitors into colonies containing immature megakaryocytes in vitro in the absence of thrombopoietin.36 The chemokine CXCL12 appears to play a role in megakaryocyte proliferation. In vitro, the chemokine acts in synergy with thrombopoietin to support the survival and proliferation of megakaryocyte progenitors.37 The combination of fibroblast growth factor (FGF)-4 and CXCL12 restores megakaryopoiesis in TPO and c-mpl null mice.38
The survival and proliferation of megakaryoblasts depend on at least two thrombopoietin-induced signaling pathways: PI3K and mitogen-activated protein kinase (MAPK). In the presence of chemical inhibitors of PI3K, the favorable effects of thrombopoietin on megakaryocyte progenitor survival and proliferation are eliminated,39 although constitutively activating this pathway is not sufficient for thrombopoietin-induced growth. MAPK is another important signaling pathway stimulated by thrombopoietin. Using purified marrow megakaryocytic progenitors and model cell lines, several groups showed that inhibition of MAPK blocks megakaryoblast maturation26,40–42 because of its effect of activating Ets transcription factors.
Stage II megakaryocytes contain a lobulated nucleus and more abundant, but less intensely basophilic, cytoplasm. Ultrastructurally, the cytoplasm contains more abundant α granules and organelles. The demarcation membrane system begins to expand at this stage of development. Stage II megakaryocytes measure up to 30 μm in diameter, constitute approximately 25 percent of marrow megakaryocytes, and are the stage of development during which endomitosis is most prominent, generating cells displaying ploidy values of 8N to 64N.
Whereas megakaryoblasts are generally thought to be able to expand by cell division, at an early stage of their maturation, the cells begin to undergo endomitosis, in which cells diverge from the normal cell cycle during mid to late anaphase. Like normally mitotic cells, endomitotic megakaryocytes condense their chromatin into chromosomes, form a spindle, dissolve the nuclear membrane, and assemble the chromosomes on a metaphase plate, then the chromosomes begin to separate during early anaphase. However, rather than the dividing chromosomes migrating to opposite poles of the cell to allow the formation of a cleavage furrow, the chromosomes quickly decondense, the nuclear membrane reforms around the entire chromosomal complement, and the endomitotic cells reenter G1 phase followed by S phase. A number of attempts to understand this process at the biochemical level have involved leukemic cell lines. Alterations in cyclin B, cdc2, cell-cycle kinase inhibitors, and aurora kinases have been claimed to be responsible for endomitosis.43,44 Unfortunately, although these hypotheses possibly explain the polyploidy in various leukemic cell lines, the hypotheses have not been substantiated in studies of normal endomitotic megakaryocytes.19,45 Endomitosis departs from a normal mitotic cell cycle at the late anaphase stage, when furrow invagination aborts short of cell abscission.46 Additional studies indicate that disordered localization of the small G-protein RhoA may be responsible for this property.46 Confirmation that a decrease in proper RhoA function is critical for endomitosis comes from the genetic elimination of RhoA from the megakaryocytic lineage; RhoA null megakaryocytes display enhanced polyploidy, although the released platelets are characterized by abnormal membrane rheology, resulting in their rapid clearance from the circulation.47 Proper RhoA localization is controlled by its activation by the RhoA guanosine triphosphate (GTP) exchange factor (GEF) ECT2; ECT2 is down-modulated during the switch from mitosis to endomitosis in megakaryocytes, providing a mechanistic explanation for the onset of endomitosis.48
Early in megakaryocyte development, the cytoplasm acquires a rich network of microfilaments and microtubules. Toward stages III and IV, the proteins accumulate in the cell periphery, creating an organelle poor peripheral zone. Biochemically, the megakaryocyte cytoskeleton is composed of actin, α-actinin, filamin, nonmuscle myosin (including the product of the MYH9 gene, mutated in several giant platelet thrombocytopenic syndromes49; Chap. 7), β1-tubulin, talin, and several other actin-binding proteins. Like platelets, megakaryocytes can respond to external stimuli by changing shape, transporting organelles around the cytoplasm, and secreting granules. These functions are dependent on the microfilament and microtubule systems of the cell. In addition, microtubules play a vital role during the later stages of platelet formation.50
As discussed earlier, GATA-1 is vital for committing primitive multipotent progenitors to the erythroid–megakaryocyte pathway. However, the transcription factor also is critical later in megakaryopoiesis, for cytoplasmic development. The first convincing evidence that GATA proteins affect megakaryocyte development came from overexpression studies of GATA-1 in a leukemic cell line, in which the transcription factor led to partial megakaryocytic differentiation.51 Reduction in GATA-1 expression also impairs cytoplasmic development in murine megakaryocytes, reducing demarcation membranes and platelet-specific granules.29 Additional transcription factors expressed during stage II megakaryocyte development include RUNX-1, Tal1, and Fli1, but these transcription factors appear to play far greater a role in megakaryocyte maturation and platelet formation and are discussed in “Stage III/IV Megakaryocytes” below.
Although more prominent in later stages of differentiation (Fig. 1–3), platelet-specific α granules first begin to form adjacent to the Golgi apparatus as 300- to 500-nm round or oval organelles in stage II megakaryocytes. Three distinct compartments are recognized in α granules: (1) a central, electron-dense nucleoid, containing fibrinogen, platelet factor-4, β-thromboglobulin, transforming growth factor (TGF)-β1, vitronectin, and tissue plasminogen activator–like plasminogen activator; (2) a peripheral zone, containing tubules and von Willebrand factor (arranged much like that seen in endothelial cell Weibel-Palade bodies); and (3) the granule membrane, containing many of the critical platelet receptors for cell rolling (P-selectin), firm adhesion (GPIb-V-IX), and aggregation (integrin αIIbβ3). Proteins present in α granules arise from de novo megakaryocyte synthesis (e.g., GPIb-V-IX, GPIV, integrin αIIbβ3, von Willebrand factor, P-selectin, β-thromboglobulin, platelet-derived growth factor), nonspecific pinocytosis of environmental proteins (albumin and immunoglobulin G), or cell surface membrane receptor-mediated uptake from the environment (e.g., fibrinogen, fibronectin, factor V). Insights into platelet granule formation have come from a molecular understanding of Hermansky-Pudlak syndrome (HPS). In this disorder, characterized by oculocutaneous albinism and a qualitative platelet bleeding disorder, a complex of at least eight proteins form in various granule-associated complexes such as the biogenesis of lysosome-related organelles complexes, which affect δ granule formation.52 These complexes are thought to be involved in cargo transport of a number of subcellular granules, such as lysosomes, melanosomes, and platelet δ granules.
Figure 1–3.
A. Ultrastructure of the cytoplasm of a mature megakaryocyte. The majority of the granules are α granules (αGr) exhibiting dense nucleoid. Demarcation membranes (DM) are slightly dilated. Transverse sections of microtubules (Mt) are dispersed. At the periphery, a longitudinal microtubule runs under the cell membrane (arrows). Dense aggregates of glycogen (Gly), small cisternae of endoplasmic reticulum (ER), and free ribosomes are seen (magnification ×30,320). B. Morphology of an α granule. Dense nucleoid is located at the top. In a clear zone at the opposite pole, four transverse sections of tubular structures are adjacent to the granule membrane (magnification ×37,200). C. Dense body can be distinguished from α granule by the black deposit when calcium is added to the fixative (magnification ×37,200). D. Cytochemical detection of acid phosphatase using β-glycophosphate as substrate and cerium as a trapping agent. Dense cerium–phosphate precipitates are present in lysosomal granules, whereas α granules are unreactive (magnification ×37,200). E. Microperoxisome visualized using alkaline diaminobenzidine. Note the small size of a reactive granule compared to the α granule. F. Distribution of a dense tracer filling the lumen of the demarcation membrane system in a maturing megakaryocyte (arrows). In contrast to the demarcation membrane system, which is open to the extracellular space, the endoplasmic reticulum (ER) is not labeled (magnification ×9700). (Used with permission of Dr. J. Breton-Gorius.)
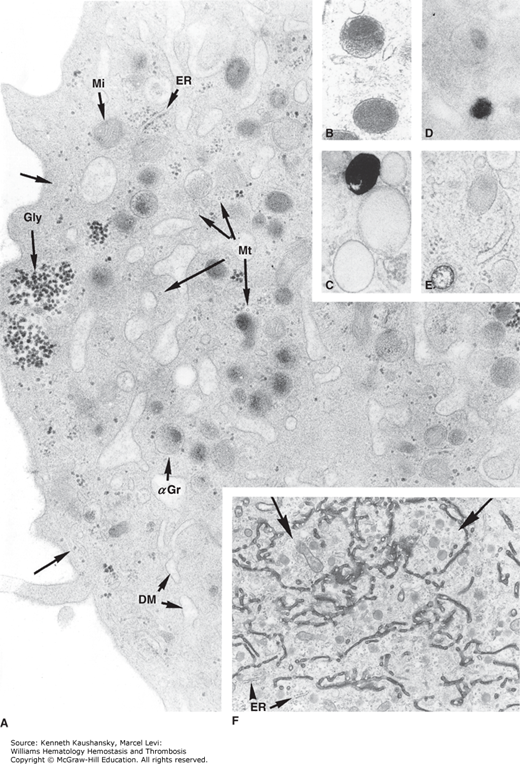
Continued cytoplasmic maturation characterizes stage III/IV megakaryocyte development (Fig. 1–4). Cells are extremely large (40 to 60 μm in diameter) and display a low nuclear-to-cytoplasmic ratio. Cytoplasmic basophilia disappears as cells progress from stage III to stage IV. The demarcation membrane system gradually replaces the endoplasmic reticulum and Golgi apparatus during the final stages of maturation. The nucleus usually is eccentrically placed. Although the nucleus sometimes appears as several distinct nuclei in biopsy sections, it remains highly lobulated but single at all stages of megakaryocyte development. In occasional marrow sections (Fig. 1–4C), neutrophils or other marrow cells are seen transiting through the cytoplasm of the mature megakaryocyte, a process termed emperipolesis, and this is of no pathologic significance.
Figure 1–4.
Megakaryocyte morphology. A. Normal human marrow biopsy. Two megakaryocytes are evident. In one case the section is through the cell at the level of the nuclei (horizontal arrow), and in the other, it is through the cytoplasm above or below the nucleus (vertical arrow). B. Normal human marrow aspirate. Mature (stage III) megakaryocyte with a multilobated nucleus and abundant cytoplasm. C. Normal human marrow aspirate. Mature megakaryocyte with a neutrophil embedded in the cytoplasm. Many ultrastructural studies have confirmed that this appearance represents marrow cells entering the canalicular system of megakaryocyte cytoplasm through its opening to the exterior of the cell (emperipolesis). (Reproduced with permission from Lichtman’s Atlas of Hematology, www.accessmedicine.com.)
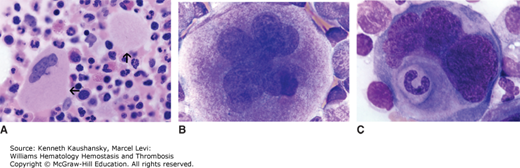
Careful microscopic studies have localized marrow megakaryocytes to the abluminal surface of sinusoidal endothelial cells. In specially prepared specimens, the megakaryocytes can be seen issuing long, slender cytoplasmic processes between endothelial cells and into the sinusoidal lumen, structures termed proplatelet processes (Fig. 1–5).53 The processes have been reproduced in vitro and in vivo.6 The processes consist of a β-tubulin cytoskeleton and highway, transporting organelles and platelet constituents from the megakaryocyte to the terminal projection, the nascent platelet.17
Figure 1–5.
Megakaryocyte proplatelet processes in the marrow sinusoid. Scanning electron micrograph showing the luminal view of the confluence of two marrow sinusoids with two proplatelet processes protruding through the lining endothelial cells. One of the processes has intermittent constrictions (arrows), indicating potential sites for platelet formation. Other cells depicted include lymphocytes and erythrocytes (magnification ×3000). (Reproduced with permission from Becker RP, De Bruyn P: The transmural passage of blood cells into myeloid sinusoids and the entry of platelets into sinusoidal circulation; a scanning electron microscope investigation. Am J Anat 1976 Feb;145(2):183–205.)
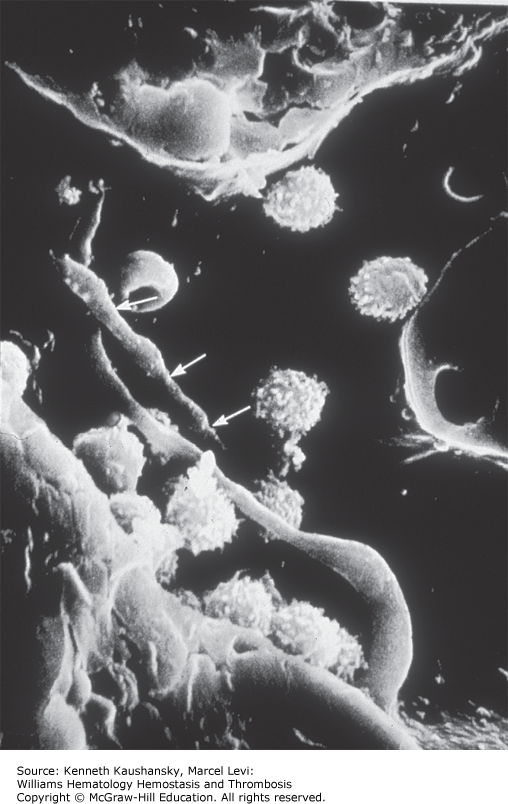
Most of the specific characteristics of platelet membranes are present at stages III and IV of megakaryocyte development. Megakaryocyte membrane lipid composition progressively changes through development, achieving approximately four times the content of phospholipids and cholesterol as found in immature cells. Megakaryocytes contain approximately the same amounts of membrane neutral lipid and phospholipid as platelets but contain relatively more phosphatidylinositol and less phosphatidylserine and arachidonic acid.
One transcription factor that plays an important role in the final stages of megakaryocyte maturation is nuclear factor-E2 (NF-E2). Initially described as an erythroid-specific, heterodimeric protein belonging to the basic leucine zipper family of transcription factors, NF-E2 is composed of a ubiquitously expressed p18 subunit, and a 45-kDa protein (p45) expressed only in erythroid cells and megakaryocytes.54 NF-E2 binds to tandem AP-1–like motifs, such as those seen in the second deoxyribonuclease (DNAse) hypersensitive site of the β-globin locus control region, and is required for β-globin expression.55 However, genetic elimination of p45 failed to significantly affect erythropoiesis. Rather, p45-deficient mice display prominent alterations in megakaryocyte development and severe thrombocytopenia,56 leading to death from widespread hemorrhage soon after birth. Examination of the animals reveals modest expansion of marrow megakaryocytes but failure of the cells to produce platelets because of defects in cytoplasmic maturation, including substantial reductions in platelet granules and demarcation membranes. Thus, the loss of either GATA-1 or NF-E2 results in failure of late aspects of cellular maturation. As p45 NF-E2 is induced by GATA-1/FOG,57 the lack of cytoplasmic development in GATA-deficient mice likely is an indirect effect. The role of transcription factors in late megakaryopoiesis has been reviewed.58
Nearly all studies of megakaryopoiesis have focused on the marrow. The final stages of megakaryocyte fragmentation also are proposed to occur in the lung, at least for some cells, a theory based on the finding that platelet levels in pulmonary venous blood exceed those found in the pulmonary artery.59 Whether this process represents the migration and fragmentation of intact megakaryocytes in the lung or merely the final size reduction of large fragments of megakaryocyte cytoplasm that also are released into the blood is not clear. Some data exist supporting the notion that lung megakaryocytes contribute to blood platelet production.60 However, in mice administered high doses of thrombopoietin, with platelet counts as high as 4 million/μm3, neither intact megakaryocytes nor denuded nuclei were found in the lungs of these animals.61 One study found that canine lungs contain 2.5 megakaryocytes per cm2.62 Extrapolation of these data suggest human lungs contain approximately 6000 megakaryocytes, only enough to account for a small proportion (<0.1 percent) of daily platelet production.
Numerous studies have indicated thrombopoietin is the primary regulator of megakaryocyte maturation.36,63 However, despite the importance of the hormone for generation of fully mature megakaryocytes from which platelets arise, elimination of the cytokine during the final stages of platelet formation is not detrimental.64 Although proplatelet formation is possible under serum-free conditions,65 most investigators have reported the presence of plasma and/or an integrin ligand-containing substratum (e.g., fibronectin or vitronectin) stimulates the process substantially.64,66 These findings suggest external signals probably are required for normal platelet formation. One report suggests the thrombin–antithrombin complex with or without high-density lipoprotein particles mediates the favorable effect of plasma on proplatelet formation,67 although other data suggest prothrombin and its conversion to thrombin by megakaryocytes inhibit the process.68 Although the cytokine(s) required for this process is not known, activation of protein kinase Cα clearly is necessary for the process to occur.66
Platelet formation involves massive reorganization of megakaryocyte cytoskeletal components, including actin and tubulin, during a highly active, motile process in which the termini of the process branch and issue platelets.5 The size of the individual platelets formed is of interest. Unfortunately, little is known about this aspect of platelet formation except that tubulin is proposed to act as a measuring device for the proper site to pinch off platelets from proplatelet processes. The mechanism of platelet formation clearly must be affected in some way by the transcription factor GATA-1, the GPIb-IX complex, the Wiskott-Aldrich syndrome protein, and platelet myosin, as defects in each of these genes leads to unusually large or small platelets (Chap. 7).69,70 Finally, localized cytoplasmic membrane proteolysis, a sublethal form of apoptosis, likely plays a role in initiating the final stages of platelet formation.71
Several cytokines, first identified using alternate hematopoietic activity assays, affect megakaryocyte development. IL-3, granulocyte-macrophage colony-stimulating factor, and stem cell factor support the proliferation of megakaryocytic progenitors in plasma-containing cultures.72–74 In 1994, several groups reported the purification and/or cloning of thrombopoietin.75 This cytokine clearly is the primary regulator of megakaryopoiesis but cannot explain thrombopoiesis in its entirety.
IL-3 is a 25- to 30-kDa protein produced almost exclusively by T lymphocytes.76 The mature human protein contains 133 amino acids, but N-linked carbohydrate modification accounts for the larger than expected Mr of the cytokine. Granulocyte-macrophage colony-stimulating factor is an 18- to 30-kDa protein also produced by T lymphocytes. However, endothelial cells, monocytes, and fibroblasts also produce the protein, and, like IL-3, granulocyte-macrophage colony-stimulating factor is highly modified with both N-linked and O-linked carbohydrate.77 Although the two proteins display essentially no primary sequence homology, their tertiary structures are highly related,78 and the receptors for the two cytokines share a common subunit.79 However, the physiologic relevance of IL-3 and granulocyte-macrophage colony-stimulating factor for steady-state thrombopoiesis is uncertain. Administration of the cytokines to mice or humans has only minimal effects on thrombopoiesis, and genetic elimination of either has no impact on megakaryopoiesis, even when combined with elimination of other thrombopoietic cytokines.80,81
IL-6, cloned by several groups using multiple assays (hepatocyte growth, myeloma cell growth, immunoglobulin secretion, antiviral activity), enhances megakaryocyte maturation. IL-6 is a 26-kDa polypeptide produced by T lymphocytes, fibroblasts, macrophages, and stromal cells in response to inflammatory stimuli.82 The mature protein is composed of 184 amino acids, contains two disulfide bonds, and displays both N-linked and O-linked carbohydrate modification. Although IL-6 alone fails to affect in vitro megakaryopoiesis, it augments the number of megakaryocyte colonies obtained in the presence of IL-3 or stem cell factor83 and exerts primarily a differentiating effect.84,85 Administration of IL-6 to mice or nonhuman primates or patients results in a modest thrombocytosis.86–88 These findings suggest IL-6 contributes to megakaryopoiesis in vivo, a conclusion supported by its production by tumor cells in selected cases of paraneoplastic thrombocytosis.89 However, genetic elimination of the cytokine fails to significantly affect basal platelet production.90 Evidence suggests the cytokine affects platelet production indirectly91 by stimulating thrombopoietin production.
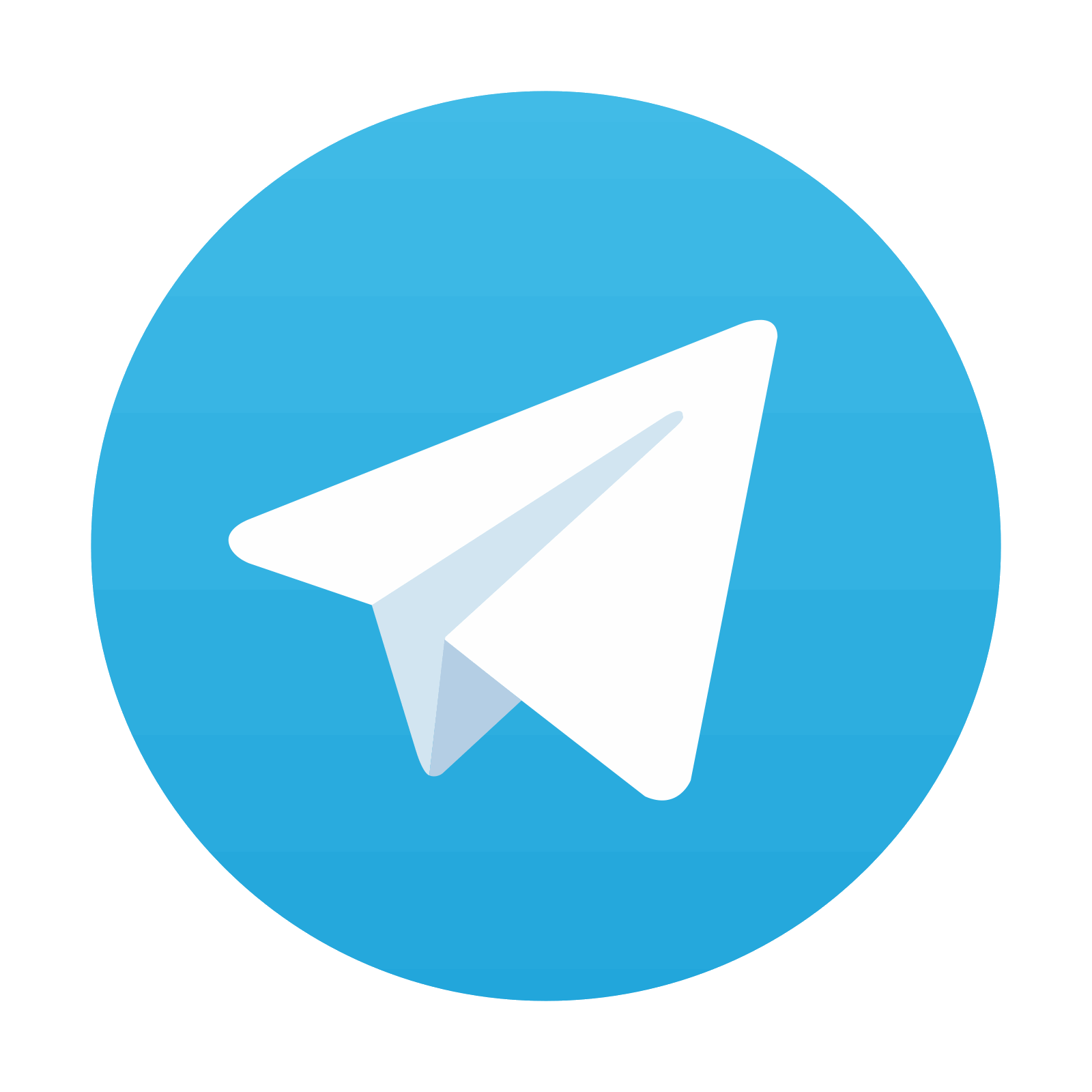
Stay updated, free articles. Join our Telegram channel
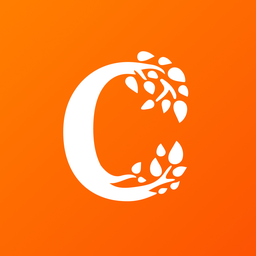
Full access? Get Clinical Tree
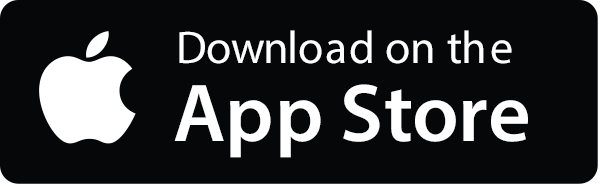
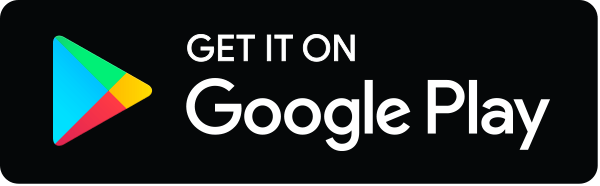