Method
Measurement
Advantages
Disadvantages
Value in drug development decisions
Mouthpieceor facemask
O2 consumption and CO2 production from breathing
Minimal response time; no dilution of respiratory gases
Mouthpiece and facemask are tolerated for limited time interval
Limited; measurement of energy expenditure for defined activities including VO2 max
Ventilated hood
O2 consumption and CO2 production from breathing
Standardised measurement conditions
Mainly applicable under resting conditions
Limited; measurement of basal metabolic rate and diet-induced energy expenditure
Respirationchamber
O2 consumption and CO2 production from breathing
Definition of living environment including diet and physical activity
High dilution of respiratory gases; confined environment
High; evaluation of effects of diet, physical activity and sleep on energy expenditure
Doublylabelledwater
CO2 production from isotope elimination as measured in urine, saliva or blood
Assessment of total energy expenditure without any interference
Minimal observation time several days or weeks, depending on the physical activity level of the subject
Moderate; evaluation of daily energy requirement and validation of reported food intake and physical activity assessment
Conclusions
Designing studies to evaluate intervention effects on energy expenditure, including drugs, should be based on the energy expenditure component as targeted. The indicated method for the measurement of maintenance expenditure is a ventilated hood. Diet-induced energy expenditure can be measured with a ventilated hood or in a respiration chamber. Activity-induced energy expenditure is measured under standardised conditions with a mouthpiece, facemask or in a respiration chamber and under free-living conditions with doubly labelled water. Energy expenditure can be estimated and evaluated with alternative methods including prediction equations for maintenance expenditure, based on height, weight, age and gender; doubly labelled water validated accelerometers to assess activity-induced energy expenditure; and measurements of food intake as evaluated with the doubly labelled water technique.
Introduction
Energy expenditure is measured primarily to assess energy requirement. Measured energy expenditure is the basis for food intake recommendations including nutritional advice and for enteral and parenteral nutrition in a clinical setting. The level of resting energy expenditure, as affected by maintenance metabolism, is a reflection of the health status of a subject. Activity-induced energy expenditure is a measure for the physical activity level. Maximal energy expenditure is a measure for physical fitness.
The design of weight-maintenance diets, as applicable in clinical settings, is based on energy requirement as derived from measured energy expenditure. Alternatively, energy requirement can be based on reported food intake. However, reported intake is often lower than habitual energy intake, resulting in weight loss when subjects are fed according to what they report they normally eat.
Measured energy expenditure is a criterion method for the evaluation of methods for the assessment of food intake and physical activity. Nowadays, the validity of reported food intake, with questionnaires, interviews or dietary records, can be evaluated with a measurement or estimate of energy expenditure under daily living conditions. The validity of methods to assess the physical activity level of a subject like questionnaires, interviews, activity records, heart rate monitoring, pedometers or accelerometers can be evaluated by measurement of daily energy expenditure in combination with a measurement or estimate of resting energy expenditure.
Studies on energy expenditure in large numbers of subjects have resulted in prediction equations based on subject characteristics, for situations preventing real measurements or usage as a reference. A well-known example is the Harris and Benedict equation for the prediction of resting energy expenditure, based on height, weight, age and gender [1]. Body weight and habitual physical activity are the main determinants of energy requirements for subjects with different lifestyles. Total energy expenditure, expressed as a multiple of resting or maintenance expenditure, is an expression of the physical activity level (PAL) of a subject. The Food and Agriculture Organization of the United Nations (FAO), World Health Organization (WHO) and United Nations University (UNU) classified lifestyles in relation to the intensity of habitual physical activity or PAL. A sedentary or light activity lifestyle has a PAL value of 1.40–1.69, an active or moderately activity lifestyle has a PAL value of 1.70–1.99 and a vigorous or vigorously activity lifestyle has a PAL value of 2.00–2.40 [2].
Nutrition and pharmacological research uses human energetics for the study of the efficacy of nutrients and drugs for body weight regulation. Then, effects are studied in relation to energy expenditure as well as substrate utilisation. Substrate utilisation, i.e. the oxidation of protein, carbohydrate and fat, can be calculated from the simultaneous measurement of oxygen consumption, carbon dioxide production and urine-nitrogen loss. Thus, it can be evaluated whether a nutrient or drug is thermogenic or affects, for instance, fat oxidation in subjects with overweight and obesity.
Background
Sanctorius (Italy, 1561–1636) started to assess energy expenditure from weight measurements. He observed that the weight of what he ate and drank was larger than the weight loss in faeces and urine. The difference was ascribed to insensible perspiration. We now know insensible perspiration is more a measure for water loss through evaporation than for energy expenditure [3]. The next step was a calorimeter, measuring the heat given off by the body. Lavoisier (Paris, 1780) placed a guinea pig in a cage surrounded by ice. As the ice melted from the animal’s body heat, the water was collected in a container. The amount of melted water was a measure for heat production. Heat exchange with the environment was prevented with an outer jacket around the inner jacket surrounding the cage, packed with ice as well. The method of measuring energy expenditure by heat loss is named direct calorimetry. Around 1900, the first direct calorimeter for humans was developed. In between, indirect calorimetry was developed, measuring energy expenditure by measuring oxygen consumption and/or carbon dioxide production. The present state of the art is assessing energy expenditure with indirect calorimetry. Measurement of gas exchange discloses more about metabolic processes.
In indirect calorimetry, energy expenditure is calculated from oxygen consumption, carbon dioxide production and urine-nitrogen loss. The method is based on the relationship between the amount of energy produced in oxidation of the energy substrates carbohydrate, fat and protein and the amount of carbon dioxide produced by this process. Protein oxidation results in carbon dioxide, water and nitrogen, where the latter is excreted in the urine. The resulting three equations with three unknowns can be solved for energy expenditure. Examples of equations for the calculation of energy expenditure derived from these figures are the Weir equation [4]:
and the Brouwer equation [5]:
Differences in the coefficients are caused by differences in assumptions on gaseous exchange and energy release from the metabolised carbohydrate, fat and protein. The contribution of measured urine-nitrogen loss to calculated energy expenditure, the so-called protein correction, is only small. In the case of a normal protein oxidation of 10–15 % of daily energy production, the protein correction for the calculation of energy expenditure is smaller than 1 %. Usually, urine-nitrogen is only measured when information on the contribution of carbohydrate, fat and protein to energy expenditure is required. For calculating the energy expenditure, the protein correction is often neglected.


Comparing indirect calorimetry with direct calorimetry provides information on work efficiency, the fraction of energy expenditure for external work. Webb et al. [6] simultaneously measured energy production and heat loss in subjects at rest, walking on a treadmill and cycling on an ergometer. Subjects stayed in a respiration chamber for measuring energy production from gaseous exchange while wearing a suit calorimeter to quantify heat loss. At rest, indirect calorimetry-assessed energy expenditure matched heat loss as measured with direct calorimetry. Resting energy expenditure was on average 100 Watt, typical for a young adult. During physical activity, heat loss was systematically lower than indirect calorimetry-assessed energy expenditure. The difference increased with walking speed and cycling load. During cycling, indirect calorimetry-assessed energy expenditure matched the sum of heat loss and power output. The work efficiency during cycling, power output divided by energy expenditure, was in the range of 15–25 %. Thus, heat loss matches total energy expenditure at rest but can be up to 25 % lower than total energy expenditure during endurance exercise.
Key Techniques
Current techniques utilising indirect calorimetry for the measurement of energy expenditure in man are a mouthpiece or facemask, ventilated hood, respiration chamber and doubly labelled water.
Mouthpiece or Facemask
Metabolic gas analysis systems with a mouthpiece or facemask are typically used to determine physical fitness in patients and athletes. Oxygen consumption and carbon dioxide production are measured on a breath-by-breath basis. The oxygen and carbon dioxide concentrations are commonly measured with a paramagnetic oxygen analyser and an infrared carbon dioxide analyser, respectively. Flow is measured with a variety of mass flow detectors. Generally, results are based on mathematical integration after reconstructing the waveforms of gas concentrations and matching the curves with the flow signal. Then, the critical aspect is the reconstruction procedure and the temporal alignment of the gas flow with the gas analysis. Alternatively, the system has a mixing chamber, providing mean values for a specified number of breaths or a specified time interval. Measurement systems have evolved from metabolic carts to portable systems for field-based studies. Before and after usage, the system is calibrated with gases of known concentration including ~16 % oxygen, 4–5 % carbon dioxide and room air, and the flow detector is checked by passing a fixed air volume with a syringe. Mouthpiece and facemask systems supply the most direct information on gaseous exchange with minimal response time. Expiration gases can be measured without dilution. Disadvantages are interference with the measurement of breathing through a mouthpiece with nose clip or facemask, also limiting the time interval of measurements.
Ventilated Hood
A ventilated hood is typically used to measure resting energy expenditure and energy expenditure for food processing or diet-induced energy expenditure. The subject lies with his head enclosed in a plastic canopy, sealed off by plastic straps around the neck. Air is sucked through the canopy with a pump and blown into a mixing chamber where a sample is taken for analysis. Measurements taken are those of the airflow and of the oxygen and carbon dioxide concentrations of the air flowing in and out. The airflow is adjusted to keep differences in oxygen and carbon dioxide concentrations between inlet and outlet within a range of 0.5–1.0 %. For adults this means airflow rates around 50 l/min. A ventilated hood allows the subject to relax completely. Discarding the first minutes of an observation interval can eliminate effects of habituation. Calibration with gases of known concentration can be performed before and after as well as during observations, by automated switching. Flow metres usually do not require frequent calibration, showing little or no drift in time. Subjects getting restless limit observation time.
Respiration Chamber
A respiration chamber is an airtight room, which is ventilated with fresh air. Basically the difference between a respiration chamber and a ventilated hood system is size. In a respiration chamber the subject is fully enclosed instead of enclosing the head only, allowing physical activity depending on the size of the chamber. With both methods, the airflow rate and the oxygen and carbon dioxide concentration difference between inlet and outlet air are measured in the same way. The flow rate to keep differences for oxygen and carbon dioxide concentrations between inlet- and outlet air in the range of 0.5–1.0 % is slightly higher in the respiration chamber than in the ventilated hood system as in the chamber subjects never lie down over the full length of an observation interval. In a sedentary adult a typical flow rate is 50–100 l/min, while in exercising subjects the flow has to be increased to over 100 l/min. In the latter situation one has to choose a compromise for the flow rate when measurements are to be continued over 24 h including active and inactive intervals. During exercise bouts the 1 % carbon dioxide level should not be surpassed for long periods. During resting bouts, like an overnight sleep, the level should not fall too far below the optimal measuring range of 0.5–1.0 %. Changing the flow rate during an observation interval reduces the accuracy of the measurements due to the response time of the system. A normal size respiration chamber has a volume of 10–30 m3 and is equipped with a bed, toilet, washbasin and communication facilities like telephone, radio, television and Internet. Basically it is a hotel room. The experimenter sets the room temperature. Food and drink is delivered through an air lock according to the experimental design. Physical activity is often monitored with a radar system to know when and how often subjects are physically active. A respiration chamber can be equipped with a cycle-ergometer or a treadmill to perform standardised workloads. A respiration chamber has a much longer response time than a ventilated hood. Though the flow rate in both systems is comparable, the volume of a respiration chamber is more than 20 times the volume of a ventilated hood. Consequently, the minimum length of an observation period in a respiration chamber is in the order of 5–10 h.
Doubly Labelled Water
The doubly labelled water technique is the most recent variant of indirect calorimetry. The doubly labelled water technique for the measurement of energy expenditure is based on the discovery that oxygen in the respiratory carbon dioxide is in isotopic equilibrium with the oxygen in body water. The technique involves enriching the body water with an isotope of oxygen and an isotope of hydrogen and then determining the washout kinetics of both isotopes. Most of the oxygen isotope is lost as water, but some is also lost as carbon dioxide because CO2 in body fluids is in isotopic equilibrium with body water due to exchange in the bicarbonate pools. The hydrogen isotope is lost as water only. Thus, the washout for the oxygen isotope is faster than for the hydrogen isotope, and the difference represents the CO2 production. The isotopes of choice are the stable, heavy, isotopes of oxygen and hydrogen, oxygen-18 (18O) and deuterium (2H), since these avoid the need to use radioactivity and can be used safely. Both isotopes naturally occur in drinking water and thus in body water. Oxygen-18 (18O) has eight protons and ten neutrons instead of the eight protons and eight neutrons found in normal oxygen (16O). Deuterium (2H) has one proton and one neutron instead of one neutron for normal hydrogen (1H). ‘Normal’ water consists largely of the lighter isotopes 1H and 16O, the natural abundance for 2H is about 150 parts per million or 150 ppm and for 18O 2,000 ppm. Enriching the body water with doubly labelled water (2H2 18O) for the measurement of energy expenditure implies an increase of the background levels as mentioned with 200–300 ppm for 18O and with 100–150 ppm for 2H. The CO2 production, calculated from the subsequent difference in elimination between the two isotopes, is a measure of energy expenditure (Fig. 7.1). In practice, the observation duration is set by the biological half-life of the isotopes as a function of the level of the energy expenditure. The minimum observation duration is about 3 days in subjects with a high energy turnover like premature infants or endurance athletes. The maximum duration is 30 days or about 4 weeks in elderly (sedentary) subjects. An observation starts by collecting a baseline sample. Then, a weighed isotope dose is administered, usually a mixture of 10 % 18O and 5 % 2H in water. For a 70 kg adult, between 100 and 150 cc water would be used. Subsequently the isotopes equilibrate with the body water and the initial sample is collected. The equilibration time is dependent on body size and energy expenditure. For an adult the equilibration time would take between 4 and 8 h. During equilibration the subject usually does not consume any food or drink. After collecting the initial sample, the subject performs routines according to the instructions of the experimenter. Body water samples (blood, saliva or urine) are collected at regular intervals until the end of the observation period. There are different sampling protocols, i.e. multi-point versus two-point method. The ideal protocol is a combination of both, taking two independent samples: at the start, the midpoint and at the end of the observation period. Thus, an independent comparison can be made within one run, calculating CO2 production from the first samples and the second samples over the first half and the second half of the observation interval. Validation studies, comparing the method with respirometry, have shown that results based on the doubly labelled water method elicit an accuracy of 1–3 % and a precision of 2–8 %. The method requires high-precision isotope ratio mass spectrometry, in order to utilise low amounts of the very expensive 18O isotope. The doubly labelled water technique gives precise and accurate information on carbon dioxide production. Converting CO2 production to energy expenditure needs information on the energy equivalent of CO2, which can be calculated with additional information on the substrate mixture being oxidised. One option is the calculation of the energy equivalent from the macronutrient composition of the diet. In energy balance, substrate intake and substrate utilisation are assumed to be identical. Doubly labelled water provides an excellent method to measure energy expenditure in unrestrained humans in their normal surroundings over a time period of 1–4 weeks.
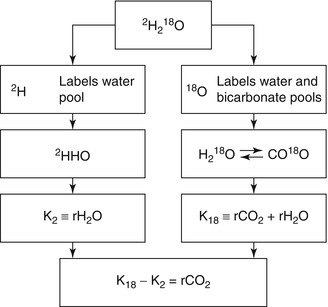
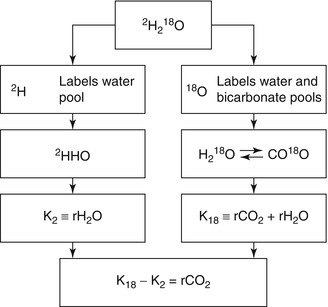
Fig. 7.1
The principle of the doubly labelled water method. The two isotopes, 18O and 2H as administered as doubly labelled water (2H2 18O), mix with the body water, where 18O exchanges with CO2 in the bicarbonate pools as well. Thus, the elimination rate of 2H (K2) is a measure for water loss (rH2O) and the elimination rate of 18O (K18) is a measure for rH2O plus carbon dioxide production (rCO2), and rCO2 = K18–K2
Validation of Methods
Calibration of indirect calorimetry methods is usually performed by calibrating the analytic procedures involved, i.e. flow detector and gas analysers or isotope ratio mass spectrometry. Independent validation of the final result should be performed as well. Indirect calorimetry systems require regular validation [7]. Mouthpiece and facemask systems are usually validated against the Douglas bag method as a gold standard [8]. For a ventilated hood and respiration chamber, alcohol combustion is a valid and easy-to-use procedure [9]. The result of the doubly labelled method has been evaluated with simultaneous respiratory gas exchange measurements in a respiration chamber [10].
The easiest and reliable method for validating indirect calorimetry is alcohol combustion. Alcohol (99.8 % methanol GR for analysis) is combusted by using a gas burner. The burner is placed on a calibrated balance connected to a computer to measure the rate of combustion during the experiment. The rate of combustion and the time interval for validation are set to mimic the oxygen consumption and carbon dioxide (CO2) production of subjects to be measured and the experimental design of the study. Mouthpiece and facemask systems can be validated indirectly by performing the test with a subject in a respiration chamber, allowing simultaneous measurement of oxygen consumption and carbon dioxide production with both systems and adopting the validated respiration chamber outcome as the criterion.
Technique Application
Indirect calorimetry methods are used to assess physical performance from energy expenditure for defined activities including VO2 max and for assessment of daily energy expenditure and its components. Here the focus is on the application for assessment of daily energy expenditure and its components. The components of daily energy expenditure are sleeping metabolic rate (SMR), energy cost of arousal, thermic effect of food or diet-induced energy expenditure (DEE) and energy cost of physical activity or activity-induced energy expenditure (AEE). Sometimes daily energy expenditure is divided into three components, taking sleeping metabolic rate and the energy cost of arousal together as energy expenditure for maintenance or basal metabolic rate (BMR). BMR usually is the main component of daily energy expenditure.
Variation of energy expenditure throughout a day, as measured in a respiration chamber, is presented in Fig. 7.2. Overnight, there generally is no food intake and, when one sleeps quietly, there is little or no physical activity. Energy expenditure gradually decreases to a daily minimum before increasing again at awakening and getting up. Then, the increase is primarily caused by activity-induced energy expenditure and subsequently by diet-induced energy expenditure as soon as one has breakfast. Thus, variation in energy expenditure throughout a day is a function of body size and body composition as determinants of SMR and BMR, physical activity as determinant of AEE and food intake as determinant of DEE.
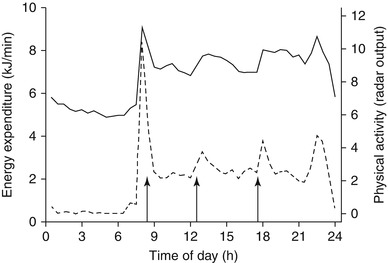
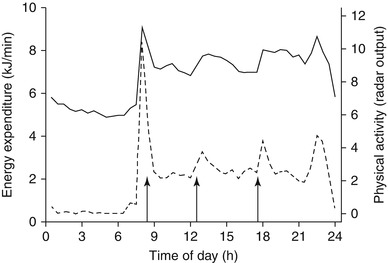
Fig. 7.2
Variation of energy expenditure (left axis, continuous line) and physical activity (right axis, broken line) throughout a day, as measured in a respiration chamber [11]. Arrows denote meal times
The indicated method for the measurement of SMR is a respiration chamber and for BMR a ventilated hood, and DEE can be measured in a respiration chamber or with a ventilated hood, as explained in the subsequent sections. Activity-induced energy expenditure can be measured under standardised conditions, as in a respiration chamber, or under free-living conditions with the doubly labelled water method. For the latter, the measurement of total energy with doubly labelled water is combined with a measurement of BMR under a ventilated hood, or alternatively, BMR is estimated with a prediction equation based on height, weight, age and gender of the subject.
Sleeping Metabolic Rate and Basal Metabolic Rate
Basal metabolic rate is defined as the daily rate of energy expenditure to maintain and preserve the integrity of vital functions. The measurement of basal metabolic rate must meet four conditions: the subject is awake, is in a thermoneutral environment to avoid heat production for the maintenance of body temperature, is fasted long enough to eliminate DEE and is at rest to eliminate AEE. For the measurement of sleeping metabolic rate, subjects must be asleep and meet the remaining three conditions for the measurement of BMR.
To perform accurate measurement of BMR, one usually adopts an inpatient protocol. A subject stays overnight in the facility, where food intake and physical activity are strictly controlled, and BMR is measured directly after waking up in the morning. A 10–12-h fast before BMR measurement is the accepted procedure to eliminate DEE. Thus, when BMR is measured at 7.00 AM, subjects should be fasted from about 8.00 PM the day before. High-intensity exercise should be prevented on the day before BMR measurement. An outpatient protocol, where subjects are transported by car or public transport to the facility after spending the night at home, produces sufficiently reproducible results when subjects are carefully instructed and behave accordingly [12].
A protocol for a BMR measurement with a ventilated hood system takes about 30 min. To eliminate effects of subject habituation to the testing procedure, the respiratory measurements over the first 10 min are discarded and the following 20 min are used to calculate BMR (Fig. 7.3). The criterion for the chosen time interval is the reproducibility of the calculated BMR value. Longer measurements tend to result in higher values because subjects become restless. It is discouraged to use a mouthpiece or facemask for collection of respiratory gases, introducing stress during breathing and resulting in an overestimate of the BMR value. Similarly, values are generally higher for subjects observed in a sitting position than for subjects in a more relaxed supine position.
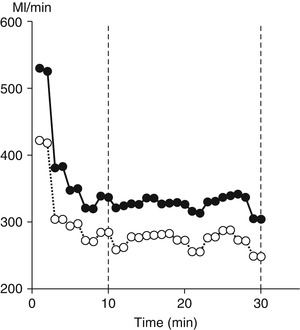
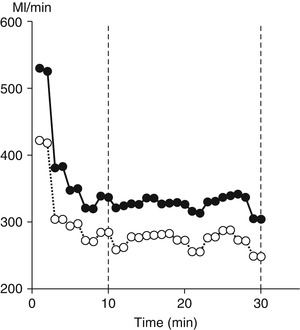
Fig. 7.3
A typical example of a basal metabolic rate measurement with a ventilated hood system, where oxygen consumption (closed dots) and carbon dioxide production (open dots) are plotted in time. To eliminate effects of subject habituation to the testing procedure, the respiratory measurements over the first 10 min are discarded and the following 20 min are used to calculate basal metabolic rate
Sleeping metabolic rate is based on a number of subsequent data points, as measured in a respiration chamber. The minimum interval for the measurement of energy expenditure in a respiration chamber, set by chamber volume and ventilation rate, is around 30 min. Then, sleeping metabolic rate is defined as the average value of six subsequent 30-min intervals with the lowest value or the lowest residual of the individual relationship between energy expenditure and physical activity over 24 h [13]. Both procedures generally result in the last 3 h of the night before waking up between 3.00 and 7.00 AM (Fig. 7.2). It is advised to measure SMR during a night following free-living conditions. Sleeping metabolic rate tends to be higher during a subsequent night of a longer stay in a respiration chamber due to increased overnight restlessness [14].
Basal metabolic rate and SMR are usually compared between subjects by standardising BMR and SMR to an estimate of metabolic body size, where fat-free mass is the main predictor. The reliable way of comparing BMR or SMR data is by regression analysis. BMR or SMR should never be divided by the absolute fat-free mass value, since the relationship between energy expenditure and fat-free mass has an axis intercept that is significantly different from zero. Comparing SMR per kg fat-free mass between women and men results in significantly higher values for women than for men. The smaller the fat-free mass, the higher the SMR/kg, and thus, the SMR per kg fat-free mass is on average higher in women with a lower fat-free mass compared to men. When fat-free mass and gender are included as covariates in a regression analysis, gender does not come out as a significant contributor to the explained variation.
In conclusion, basal metabolic rate or sleeping metabolic rate measurements should meet the conditions of being awake or asleep, respectively, being post absorptive, at rest and in the thermoneutral zone. Measured values can be compared between subjects in a regression analysis with fat-free mass and fat mass as covariates.
Diet-Induced Energy Expenditure
Diet-induced energy expenditure (DEE) is the energy expenditure for intestinal absorption of nutrients, the initial steps of their metabolism and the storage of the absorbed but not immediately oxidised nutrients. It is measured as the increase in energy expenditure above the basal fasting level after consumption of a meal, defined as the increase in energy expenditure above the basal fasting level divided by the energy content of the food ingested and commonly expressed as a percentage.
The experimental design of most studies on DEE is a measurement of resting energy expenditure before and after a test meal, with a ventilated hood system. The observation is started after an overnight fast, where subjects are refrained from eating after the last meal at 8.00 PM at the latest. Thus, with observations starting between 8.00 and 9.00 h AM the next morning, the fasting interval is at least 12 h. Postprandial measurements are made for several hours while subjects have to remain stationery, most often in a supine position, for the duration of the measurements. In some studies, measurements are 30 min with 15-min intervals allowing, e.g. for sanitary activities. A study in 131 different subjects, measured for 6 h after a breakfast covering 25 % of usual intake, indicated that DEE lasted beyond 6 h for the majority of subjects [15].
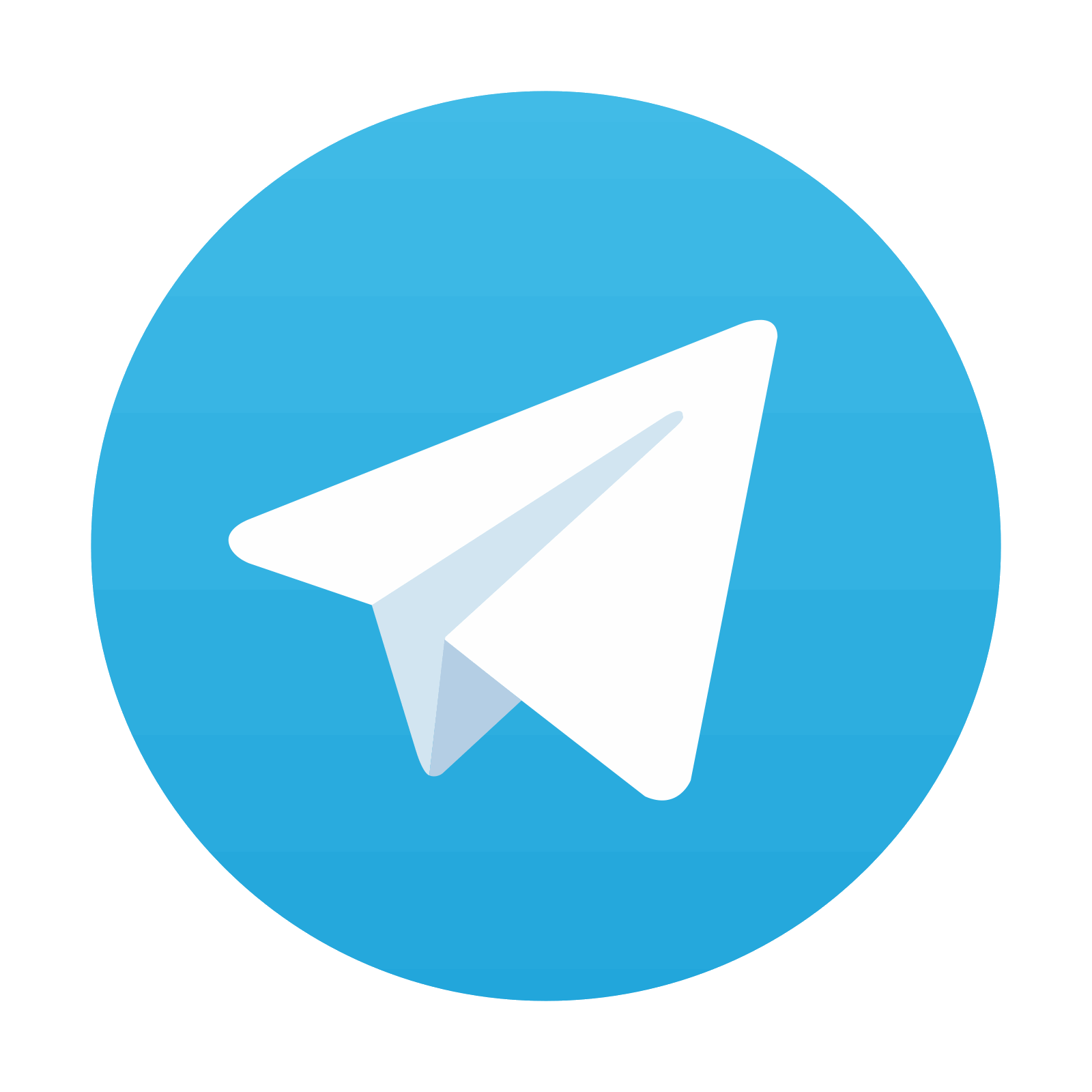
Stay updated, free articles. Join our Telegram channel
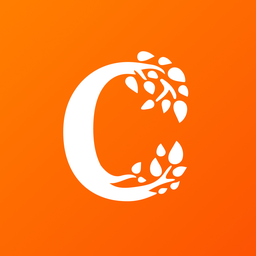
Full access? Get Clinical Tree
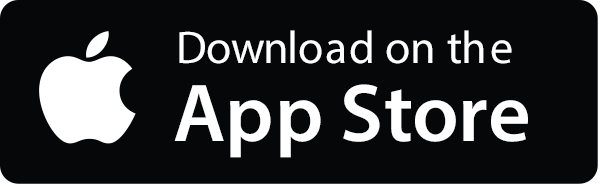
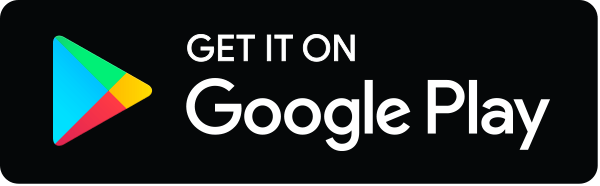