CHAPTER 4 Main Dietary Components
By the end of this chapter you should be able to:
• describe structure and associated functions of carbohydrates, fats and protein
• summarize the main features of aerobic and anaerobic metabolism of carbohydrate, fat and protein
• explain the regulation of metabolism of these major dietary components
• explain mechanisms for integration of fat, carbohydrate and protein metabolism, and response to specific dietary circumstances
• explain the glycaemic index in the context of health, with reference to specific foods
• discuss the health effects of dietary fibre
• explain what is meant by nitrogen balance
• describe methods for determining protein requirements
• describe what is meant by water balance, how this is usually maintained and the effects of imbalance
4.1 INTRODUCTION
The human body has a remarkable ability to adapt to different dietary circumstances. This is because there are biochemical mechanisms in place to ensure that flux through particular metabolic pathways is the most appropriate for the circumstances at the time. Thus, following a meal there is likely to be a drive towards the storage of fuel, as carbohydrate or as fat, whilst during the overnight fast or during the period between meals the body can mobilize these stores to ensure that all cells receive the fuel they need for normal cellular function. For these processes to occur efficiently there needs to be communication between metabolic pathways occurring in different organs but also interaction between different pathways of metabolism of the major macronutrients. For convenience this chapter examines carbohydrate, fat and protein separately, but the reader should always bear in mind that these pathways have common intermediates and there is considerable interaction between the various pathways.
4.2 CARBOHYDRATE AND ITS METABOLISM
In Western diet carbohydrate contributes about 40% to energy intake, compared with 75% or more in developing countries. Carbohydrate can be classified according to the length of the constituent polysaccharide chain. Monosaccharides are single sugar units; they exist in one of two chemical forms: aldoses, which contain an aldehyde group, as in glucose and galactose; and ketoses, containing a ketone group, as in fructose (Fig 4.1). Monosaccharides can form chemical bonds with one another, to form disaccharides. Sucrose, which is commonly known as table sugar, consists of glucose bound to fructose; lactose, which is found in milk, consists of glucose bound to galactose, and maltose, which is found in sprouting grain, consists of two glucose units. The term oligosaccharide can be used for sugars with 3–9 monosaccharide units, and this group includes maltodextrins and fructo-oligosaccharides. Polysaccharides consist of more than 9 monosaccharide units joined together. This group of sugars includes starch and non-starch polysaccharides.
Dietary carbohydrate can also be classified by the glycaemic index (GI), which is a measure of the extent to which blood glucose concentration is raised compared with an equivalent amount of a reference carbohydrate (glucose or white bread). Carbohydrate with a high GI generally provokes a higher secretion of insulin than carbohydrate with a low glycaemic index. The main glycaemic carbohydrates are glucose, fructose, sucrose, lactose and starch. Foods with a low GI generally have a high RS content, but some foods, such as bread and cornflakes, have quite a high GI despite having a high RS content.
The metabolism of glycaemic carbohydrates
Figure 4.2 gives an overview of carbohydrate metabolism integrated with fat metabolism. The first stage is glycolysis, which is the anaerobic oxidation of glucose to pyruvic acid (pyruvate), and takes place in the cytoplasm of all cells. Glycolysis yields a very modest amount of energy but because it can occur in the absence of oxygen it is important in muscle during intense exercise. Pyruvate formed from glycolysis can be oxidized further by crossing into the mitochondria for entry into the tricarboxylic acid (TCA) cycle, which generates much more energy than glycolysis. The glycolytic pathway also provides a route for the metabolism of fructose, galactose and glycerol. The metabolism of galactose and fructose occurs in the intestinal mucosa and liver, so little fructose or galactose reaches the peripheral circulation. In cells lacking mitochondria, such as red blood cells, or when conditions in a cell become anaerobic (such as in an intensely active muscle), pyruvate is reduced to lactate, which leaves the cell and goes to the liver where it can be reconverted to pyruvate. The pentose phosphate pathway (also known as the hexose monophosphate shunt) is an alternative cytoplasmic pathway for the anaerobic oxidation of glucose. This is important because it yields useful products such as pentoses for RNA and DNA synthesis, and the reduced form of the important compound nicotinamide adenine dinucleotide phosphate (NADPH), which is essential for fatty acid synthesis.
Glycogen as a carbohydrate store
Glycogen is a branched polymer of glucose. In the fed state, glycogen is synthesized from glucose in both liver (50–150 g) and muscle (350–400 g), through the stepwise addition of glucose to an existing glycogen molecule. The branched structure of glycogen means that it binds a lot of water within the molecule. In the early stages of food restriction, such as during an overnight fast, or between meals, the body uses glycogen as a fuel source (Fig 4.3), and there is an associated excretion of this bound water. This leads to an initial high rate of weight loss but it cannot be sustained because once glycogen has been depleted the rapid loss of water (and weight) will cease. In the fasting state, glycogen is broken down in the muscle and the liver. In the liver this process leads to the formation of glucose, which can be exported to other tissues. In contrast the muscle is unable to carry out the final step in the breakdown of glycogen to glucose, meaning that muscle is unable to export glucose for use by other tissues but can use the phosphorylated glucose it generates in glycolysis.
Gluconeogenesis
Cells in the liver and kidney medulla are able to synthesize glucose in a process called gluconeogenesis. This becomes particularly important during times of fasting or on very low carbohydrate diets. This process is largely a reversal of glycolysis except for three irreversible steps in glycolysis, for which alternative reactions occur. Many of the products of amino acid metabolism can be used for gluconeogenesis, since they are sources of pyruvate or intermediates in the tricarboxylic acid cycle. Substrates that give rise to acetyl CoA directly (alcohol, fatty acids, ketone bodies and ketogenic amino acids) cannot be substrates for gluconeogenesis, but glycerol, which is produced from the hydrolysis of triacylglycerol in the fasting state, can (Fig 4.3).
The control of carbohydrate metabolism
Hormonal control of carbohydrate metabolism in the fasting state
In the fasting state (sometimes known as the post-absorptive state, since it begins about 4–5 hours after a meal, when the products of digestion have been absorbed) metabolic fuels enter the circulation from the reserves of glycogen, triacylglycerol and protein laid down in the fed state. The metabolic problem in the fasting state is that the brain is largely dependent on glucose as its metabolic fuel, and red blood cells cannot utilize any metabolic fuel other than glucose. As the concentration of glucose and amino acids in the portal blood falls, so the secretion of insulin by the pancreas decreases and the secretion of glucagon increases. Glucagon has two main actions:
• stimulation of the breakdown of liver glycogen
• stimulation of the synthesis of glucose from amino acids in liver and kidney.
At the same time, the reduced secretion of insulin results in:
• a reduced rate of glucose uptake into muscle and adipose tissue
• a reduced rate of protein synthesis, so that the amino acids arising from protein catabolism are available for gluconeogenesis
• relief of the inhibition of lipid breakdown in adipose tissue, leading to release of free (non-esterified) fatty acids into the circulation.
Carbohydrate malabsorption
In certain individuals malabsorption of dietary carbohydrates may cause excessive delivery of fermentable substrate to the large intestine, causing intolerance. Congenital sucrase deficiency is a rare cause of sucrose malabsorption, which can cause gastrointestinal complaints, including diarrhoea, when an increasing amount of sucrose is introduced in the diet. Similarly, congenital lactase deficiency is a rare condition causing severe diarrhoea and malnutrition in the newborn. Breast milk has a high content (7%) of lactose.
Carbohydrate and disease
• Carbohydrates constitute the main energy source in the diet
• The main monosaccharides are all metabolized to pyruvate, or lactate under anaerobic conditions
• Pyruvate can be converted to acetyl CoA which can be oxidized further in the TCA cycle or used in the synthesis of fatty acids
• The main storage carbohydrate in the body is glycogen; its synthesis in the fed state and utilization in the fasting state are closely regulated by insulin and glucagon
• The brain has a particular requirement for glucose, and during fasting glucose can be synthesized from non-carbohydrate precursors
• The glycaemic index denotes the potential of a food to elevate blood glucose. Low glycaemic index foods may confer benefits in metabolic control of diabetes
• Carbohydrates that are not digested in the small intestine are substrates for fermentation in the colon, with probable health benefits
4.3 FAT AND ITS METABOLISM
Introduction
The main form of dietary fat is triacyglycerol (TAG) in which three fatty acids are bound to a molecule of glycerol (Fig 4.4). It is in this form that fat is stored, predominantly in adipose tissue. Phospholipids are similar in structure, but have a phosphate group and an additional base (choline, inositol, serine) in place of one of the fatty acids. Together with cholesterol, phospholipids are important constituents of cell membranes. Fatty acids consist of hydrocarbon chains with a carboxylic acid group at the head. Typically a fatty acid has between 12 and 22 carbon atoms and may contain no double bonds (saturated fatty acid) or one (monounsaturated) or more (polyunsaturated) double bonds (Fig 4.5). The hydrogen atoms at the carbon–carbon double bond may be on the same side of the double bond (cis) or on opposite sides (trans) (Fig 4.6). Trans fatty acids are absent from natural plant lipids but may be found in some animal fats such as milk fat. Trans fatty acids may also be formed during the processing of some fats for incorporation into foods. High intakes of trans fatty acids have been associated with an increased risk of cardiovascular disease.
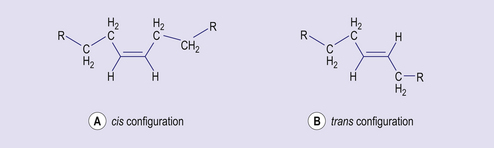
Figure 4.6 Distribution of atoms at a carbon–carbon double bond: (A) cis and (B) trans configuration.
Functions of dietary fat
Fat plays diverse roles in human nutrition. In addition to its importance as a source of energy, both for immediate utilization by the body and as a store for later utilization when food intake is reduced, dietary fat acts as a vehicle for the absorption of fat-soluble vitamins. Phospholipids and cholesterol are components of cell membranes and cholesterol is the precursor for synthesis of adrenocorticoid and sex hormones. Long chain (C20 and C22) polyunsaturated fatty acids in membrane phospholipids are the precursors of prostaglandins and other eicosanoids, compounds that have important local hormone effects.
Membrane fats in cell signalling
Various lipids are involved in cell signalling and the conversion of extracellular signals into intracellular ones. Membrane phospholipids are important mediators of hormone and neurotransmitter action and as such are involved in regulation of cellular processes such as smooth muscle contraction, glycogen metabolism and cell proliferation and differentiation. Membrane sphingolipids are important modulators of cell growth and death, and of inflammatory responses.