Fig. 11.1
Lipid structure representations. Examples of structures from the major classes of biological lipids (cholesterol, free fatty acid, triglyceride, phospholipid) are shown
Living cells acquire fatty acids for their metabolic demands from two major sources, exogenous dietary and de novo endogenous synthesis (Fig. 11.2). Proliferative embryonic cells actively use de novo synthesized fatty acids, whereas most adult normal cells (with the exception of the liver and lactating mammary gland) preferentially use exogenous fatty acids. Fatty acids are present in the diet as triglycerides, and following dietary intake, these are packaged in the intestinal epithelium into chylomicrons (Fig. 11.3). These chylomicrons are then secreted into the lymphatic system and enter the circulation via the thoracic duct. Lipolysis of these particles, initially in tissues such as the heart and lungs by the enzyme lipoprotein lipase (LPL), produces free fatty acids (FFA) that quickly associate with serum albumin. Remnant particles return to the liver where their triglycerides are assembled with apolipoprotein B 100 (apoB) for secretion as very low-density lipoprotein (VLDL) particles. In the circulation, lipolysis of VLDL produces LDL, which in turn is taken up by peripheral tissues, whereas FFA uptake is mediated by fatty acid translocase/CD36 (Figs. 11.2 and 11.3).
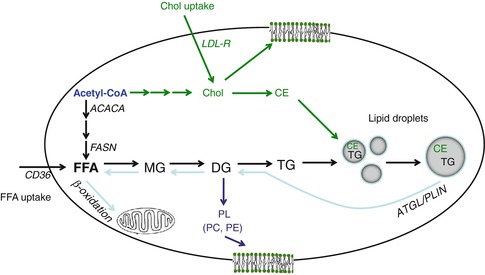
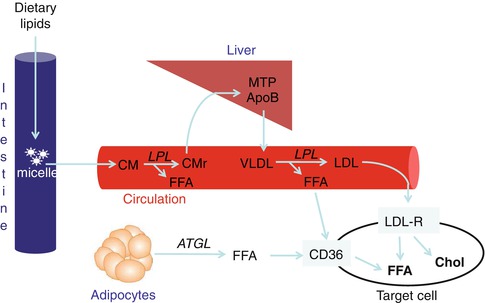
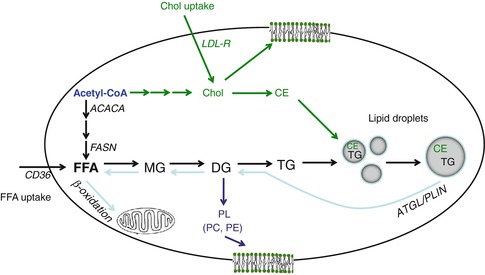
Fig. 11.2
Summary of cellular lipogenesis using endogenous and exogenous substrates. Free fatty acid (FFA) is obtained from two pathways, de novo synthesis and FFA uptake. Fatty acid is synthesized from acetyl-CoA by multiple enzymes including acetyl-CoA carboxylase (ACACA) and fatty acid synthase (FASN). FFA uptake is mediated by fatty acid translocase/CD36. FFA is used for either energy production via β-oxidation or complex lipid synthesis such as monoglyceride (MG), diglyceride (DG), and phospholipids (PL), primarily phosphatidylcholine (PC) and phosphatidylethanolamine (PE). To avoid lipotoxicity, excess FFA must be converted to triglyceride (TG), which is then incorporated into lipid droplets. Cholesterol (Chol) is derived from both acetyl-CoA and uptake through the LDL receptor (LDL-R). Cholesterol forms part of the plasma membrane, but excess cholesterol needs to be esterified into cholesterol ester (CE), which is then incorporated into lipid droplets (shaded circles). When cells require energy generation from reserved lipid stores, these can be released through lipolysis. This process is regulated by adipose triglyceride lipase (ATGL) and the PAT protein perilipin (PLIN)
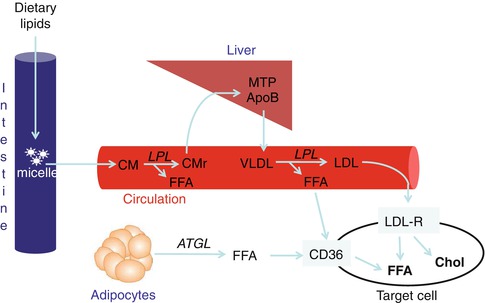
Fig. 11.3
Uptake of dietary lipids. Following dietary intake, lipids are packaged in the intestinal epithelium into chylomicrons (CM), which enter the circulation. Lipoprotein lipase (LPL) converts CM into chylomicron remnants (CMr), releasing free fatty acid (FFA). CMr are cleared by the liver where their triglycerides are assembled with apolipoprotein B 100 (apoB) by microsomal triglyceride transfer protein (MTP) for secretion as very low-density lipoproteins (VLDL) particles. In the circulation, lipolysis of VLDL by LPL produces FFA and low-density lipoproteins (LDL), which in turn are taken up by peripheral tissues via fatty acid translocase/CD36 and the LDL receptor (LDL-R), respectively. LDL is rich in FFA and cholesterol (Chol); hence, an uptake by LDL-R increases both cellular FFA and cholesterol. Additionally, FFA can be obtained from lipolysis of adipocyte lipid stores, facilitated by adipose triglyceride lipase (ATGL), which are then mobilized to target cells for uptake by CD36
In tissues capable of de novo lipogenesis, FFA are also synthesized from the precursor acetyl-CoA by multiple enzymes including acetyl-CoA carboxylase (ACACA) and fatty acid synthase (FASN) (Fig. 11.2). Cholesterol can also be synthesized from acetyl-CoA, as well as taken up through the LDL receptor (LDL-R). FFA are used either for energy production via β-oxidation or for the synthesis of complex lipids such as monoglycerides, diglycerides, and phospholipids (Fig. 11.2). As FFA are toxic to cells, excess FFA must be converted to triglyceride, which is then incorporated into lipid storage organelles, known as lipid droplets (Fig. 11.2). Similarly, excess cholesterol must be esterified into cholesterol ester (CE), which is also incorporated into lipid droplets. Lipid droplets commonly consist of a core of neutral lipids surrounded by phospholipid monolayer and associated proteins and vary greatly in size [8, 12]. Small lipid droplets represent reservoirs that can be rapidly accessed. Conversely, it is more efficient to store lipid in large versus small lipid droplets, and hence, adipose cells contain a single unilocular lipid droplet for maximum storage efficiency [12]. When cells require energy generation from reserved lipid stores, these can be released through lipolysis. This process is regulated by adipose triglyceride lipase (ATGL) and the PAT protein perilipin (PLIN) (Fig. 11.2).
Lipids in Normal Breast Biology
Mammalian infants are typically born after long gestation periods, yet remain highly reliant on their mothers after birth. As lipids represent the most dense source of energy, lipids in milk are vital to drive rapid neonatal growth and are particularly required for postnatal brain development. Milk lipid composition is the most variable attribute of milk and is affected by animal genetics, physiology, and the environment [13]. Lipid-rich milk therefore promotes neonate growth, progressively reducing neonate dependency and promoting survival. Breast-feeding human infants is known to avert serious health problems in neonates, children, and adults, leading to huge savings in medical costs [14]. The regulation of milk fat composition in ruminants is also of major economic significance, both in terms of livestock breeding and in improving the quality of milk available to consumers [13].
The mammary gland largely develops postnatally, undergoing proliferation under the influence of ovarian hormones during puberty, but then remaining largely quiescent until pregnancy. During this time, the epithelial ductal tree expands into the mammary fat pad and undergoes further branching. Alveolae develop and begin to produce milk during the late stage of pregnancy. Lipids are both taken up from the circulation and synthesized within breast epithelial cells, packaged into lipid droplets, and then released into the alveolar lumen as bilayer membrane-coated structures called milk fat globules [15]. Despite the medical and economic importance of milk in human and other species, milk composition and its regulation remain incompletely understood [14].
Lipids in Breast Cancer
As breast epithelial cells take up, synthesize, and secrete lipids during late pregnancy and lactation, it is not surprising that breast cancer is one of many cancers characterized by a lipogenic phenotype. Similar to embryonic cells, the breast and other types of cancer cells endogenously synthesize 95 % of fatty acids, despite the abundance of extracellular fatty acids available to them [16–18]. Cancer cells are highly dependent on de novo lipogenesis for their proliferation, and the lipogenic pathway is activated at a relatively early stage in various types of tumors [19]. The majority of newly synthesized fatty acids in cancer cells are converted predominantly to phospholipids and then incorporated into membrane lipids by proliferating cancer cells. It has been recently suggested that activation of de novo lipid synthesis in cancer cells leads to increased incorporation of saturated fatty acids into cell membranes, which in turn protects cells from both endogenous and exogenous damage [20]. Altered membrane properties occurring in response to de novo lipogenesis may also influence the uptake and activity of chemotherapeutic drugs in cancer cells [20].
Early Studies Demonstrating a Lipogenic Phenotype in Breast Cancer
Efforts to study biochemical alteration of breast cancer were initiated over 40 years ago. In 1966, Rees et al. investigated the lipid composition of mammary glands and mammary carcinomas from rats in various hormonal states using thin-layer chromatography (TLC) and gas–liquid chromatography [21]. Although they could identify triglyceride and phospholipid profiles in the tissues investigated, quantification of lipid species was limited to percentages of total lipids [21]. The limitations of TLC also challenged Hilf et al. in 1970 when comparing lipids in human breast cancer and normal breast tissue [22]. Although they were able to identify differences in cholesterol, FFA, triglycerides, and cholesterol esters in infiltrating ductal carcinomas compared to normal breast tissue, it was unclear which species of lipids were uniquely altered [22]. Nevertheless, they found that cholesterol, FFA, and cholesterol esters were increased in breast cancer, while triglyceride levels were decreased [22]. Sakai et al. reported similar findings, with additional data on the fatty acid composition of phospholipids and triglycerides [23]. The fatty acid compositions of phospholipids were significantly different between human breast cancer and noncancerous excised breast tissues [23]. Specifically, the proportion of monounsaturated (oleate 18:1) and polyunsaturated (docohexanoate 22:6n-3) fatty acids in the major phospholipids was significantly higher in cancer compared to noncancerous tissues [23].
Molecular Basis of Lipogenesis in Breast Cancer Cells
Lipids can either be obtained through the diet or synthesized within cells (Fig. 11.2). The processes of lipid uptake, synthesis, and subsequent metabolism are regulated by numerous transporters and enzymes (Fig. 11.2), the discussion of most of which is beyond the scope of this review. Since the expression of a vast number of proteins is deregulated in cancer cells through genetic, transcriptional, and posttranscriptional mechanisms, it would not be unexpected if some regulators of lipid uptake, synthesis, and metabolism would be thus affected, if only by chance. However, if these deregulated processes provide an advantage to the cancer cell, they will be selected for within the highly competitive environment of cancer tissue. It is now recognized that metabolic deregulation is a hallmark of cancer [24] and that changes in the expression and function of key lipogenic enzymes is actively selected for during tumorigenesis.
In cancer cells, increased glucose uptake results in increased conversion of pyruvate to acetyl-CoA in the mitochondria. Acetyl-CoA is then incorporated into the tricarboxylic acid cycle, which produces citrate in the presence of ATP. Accumulated citrate is exported to the cytoplasm where it is converted by ATP-citrate lyase (ACLY) to generate cytosolic acetyl-CoA, the precursor for FFA synthesis (Fig. 11.2). Acetyl-CoA is then carboxylated by acetyl-CoA carboxylase (ACACA) to synthesize malonyl-CoA, which is then converted to palmitate by fatty acid synthase (FASN) [19]. The unbiased analysis of large numbers of genes and proteins through genomics or proteomics approaches, respectively, has made it increasingly apparent that ACACA, ACLY, and FASN play key roles in tumor progression (Fig. 11.2). Of these three proteins, the expression of FASN and its role in mediating tumor growth has been most heavily investigated, as will be discussed in the following section.
Fatty Acid Synthase (FASN)
Increased FASN expression, relative to normal tissue, has been documented in tumors of the prostate, breast, colon, ovary, endometrium, bladder, and lung [25]. Additionally, FASN overexpression has been noted in melanoma, retinoblastoma, and soft tissue sarcoma [25–27]. FASN overexpression is primarily regulated at the transcriptional level in tumors following oncogene activation, tumor suppressor loss, or growth factor stimulation [28]. FASN levels can also be modulated by posttranslational modification and gene duplication [29, 30]. The expression levels of FASN are highest in metastatic tumors, correlate with decreased survival, and are predictive of poor outcome and disease recurrence in several tumor types [31–34]. These data suggest that FASN not only provides a metabolic advantage that may drive tumor cell survival and proliferation but may also promote a more aggressive tumor phenotype.
In normal physiology, fatty acid synthesis is crucial for development, as mice with the homozygous deletion of Fasn display an embryonic lethal phenotype [35]. On the other hand, with the exception of the liver, adipose tissue, and lactating mammary gland, FASN is expressed at low or undetectable levels in most normal adult tissues [25]. Therefore, unlike in cancer cells, fatty acid synthesis does not seem to be required for normal adult tissue maintenance. Accordingly, mice harboring liver-specific deletions of Fasn display normal liver function and no obvious phenotype, as long as they are maintained on a normal diet [36].
Coincident with the differences in FASN expression between normal and tumor tissues, there also seem to be mechanistic differences in how fatty acids are used in normal and tumor cells. In the liver and adipose tissue, fatty acids are synthesized in response to excess caloric intake. These fatty acids primarily partition toward triglyceride synthesis for fat storage. In contrast, tumor FASN-derived fatty acids preferentially partition into phospholipids that segregate into the plasma membrane or lipid rafts [37]. Additionally, it has been hypothesized that FASN also contributes to the redox status of tumor cells through oxidation of NADPH during the fatty acid synthesis cycle [38]. When all factors are taken into account, it is likely that FASN and fatty acid synthesis provide substrates to affect multiple cellular functions which support a proliferative phenotype.
ERBB2 Signalling and Lipogenesis
ERBB2 (HER2/neu) is a member of the epidermal growth factor receptor (EGFR) family of receptor tyrosine kinases that regulates biological functions ranging from cellular proliferation to transformation, differentiation, motility, and apoptosis. ERBB2 expression levels must be tightly controlled to ensure normal cellular function [39]. In vitro and in vivo studies clearly demonstrate that deregulated ERBB2 expression and activity play a pivotal role in oncogenic transformation, tumorigenesis, and metastasis [40–44]. In breast cancer, amplification of the ERBB2 gene is associated with poor prognosis, shorter relapse time, and low survival rate [40–44].
Aberrant expression of ERBB2 can trigger the activation of multiple downstream signalling pathways, including the phosphatidylinositol 3′-kinase (PI3K)/PTEN/AKT pathway and the Ras/Raf/mitogen-activated protein kinase (MAPK) pathway. These pathways induce cell proliferation and differentiation, decrease apoptosis, and/or enhance tumor cell motility and angiogenesis. Despite the recognized association of ERBB2 and these signalling pathways, less has been known about the specific effectors regulated by ERBB2 that ultimately contribute to its oncogenic effects. The use of transcriptomic analyses to identify genes that were differentially expressed in response to exogenous ERBB2 expression in breast epithelial cells identified increased FASN transcript and protein levels [45]. Similarly, in a panel of human breast cancer cell lines endogenously expressing different levels of ERBB2 and FASN, high levels of both FASN protein expression and FASN enzymatic activity were found to positively correlate with both ERBB2 amplification and ERBB2 protein overexpression [46]. A proteomic study further revealed that proteins involved in glycolysis and de novo lipogenesis pathways were highly expressed in ERBB2-positive breast carcinomas [47], supporting the notion that ERBB2-driven oncogenesis depends upon the lipogenic phenotype [19]. Additionally, mouse NIH-3T3 fibroblasts and human breast epithelial MCF10A cells engineered to overexpress ERBB2 exhibited a significant upregulation of FASN transcript and protein levels [48]. Increased FASN protein levels were also reported to be significantly higher in ERBB2-positive invasive breast tumors examined in tissue microarray format [49].
Control of endogenous FASN levels occurs through modulation of the expression and/or maturation status of the transcription factor sterol regulatory element-binding protein-1c (SREBP-1c). In ERBB2-overexpressing tumor cells, SREBP-1c expression and activation is driven by constitutive activation of the P13K/AKT and/or MAPK/ERK1/2 pathways [19]. Supporting this notion, pharmacological inhibitors of PI3K and MAPK downregulate SREBP-1c and decrease FASN transcription, ultimately reducing lipogenesis in ERBB2-overexpressing cancer cells [50]. FASN overexpression by ERBB2-mediated oncogenic stimuli can also be abrogated by deletion of the major SREBP-binding site from the FASN promoter [51].
An alternative mechanism for ERBB2-FASN induction has also been proposed by Yoon et al [52]. They reported that the induction of FASN in ERBB2-overexpressing breast cancer cells was neither accompanied by changes in FASN transcript levels nor was mediated by the activation of SREBP-1c. Rather, the 5′- and 3′-untranslated regions of FASN mRNAs appeared to be involved in selective FASN translational induction that was mediated by the mammalian target of rapamycin (m-TOR)-regulated signal transduction. In this translational mechanism of FASN regulation, the activation of mTOR significantly increased the synthetic rate of FASN, whereas ERBB2-induced upregulation of FASN protein expression was inhibited by both the PI3K inhibitor LY294002 and the mTOR inhibitor rapamycin [52]. These observations suggest that ERBB2-driven FASN overexpression can be regulated at multiple levels.
Gene Amplification of Lipogenic Genes
Gene amplification is a frequently employed mechanism which increases the expression of targeted genes. Classical cytogenetics approaches first identified genomic regions which were subjected to increased copy number, and then the advent of comparative genomic hybridization (CGH) considerably facilitated genomic copy number studies [53]. Array-based CGH and copy number analyses using single-nucleotide polymorphism profiling have largely superseded classical CGH, and next-generation sequencing is playing an increasingly significant role in identifying, quantifying, and physically mapping copy number changes in cancer and other cell types [54, 55]. Whereas many genes may be affected by copy number changes, only a proportion of these are likely to contribute to the cancer phenotype and represent gene amplification targets.
Genomic profiling and other approaches have shown that genes encoding key enzymes within the lipogenic pathway are increased in copy number and/or overexpressed in breast cancer. As described above, the oncogene ERBB2 located at chromosome 17q (35.1 MB) is amplified in approximately 15 % of breast cancer cases [56] and increases lipogenesis within cancer cells, at least in part by regulating FASN expression and function (see the section “ERBB2 Signalling and Lipogenes”).
It is striking that genes coding for three key enzymes of the fatty acid biosynthetic pathway also reside on human chromosome 17q, namely, FASN (77.6 MB), ACACA (32.7 MB), and ACLY (37.3 MB) [51, 57–59]. A number of these and other lipogenic genes cluster at chromosome 17q12-q21 within 5 MB of each other (Fig. 11.4) and could be commonly affected by copy number increases [60]. In contrast, FASN lies toward the telomeric end of chromosome 17q and does not form part of the lipogenic gene cluster around ERBB2 (see Fig. 11.4). To date, only one study has evaluated the correlation of FASN expression with gene copy number alterations in cancer cells. Using fluorescence in situ hybridization analysis in paraffin-embedded tissue microarrays, a significant increase in FASN copy number was found in a proportion of prostate adenocarcinomas and metastases, which was associated with increased FASN protein detection [30]. It is as yet unclear whether increased FASN copy number plays a significant role in driving increased FASN levels in breast cancer cells.
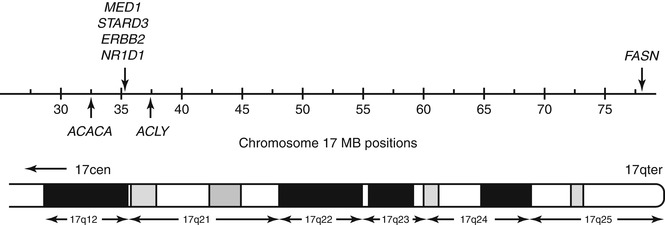
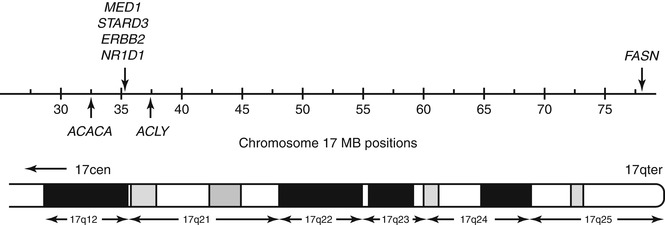
Fig. 11.4
Positions of chromosome 17q genes with known roles in lipogenesis (shown using hg 18 chromosome 17 coordinates, in MB), with the corresponding cytogenetic bands indicated on the lower ideogram. Approximate positions of genes are shown using vertical arrows: ACACA, 32.7 MB; ACLY, 37.3 MB; MED1 (previously known as PPAR γ-binding protein), 34.8 MB; STARD3 and ERBB2, 35.1 MB; N1RD1, 35.5 MB; and FASN, 77.6 MB. All genes except FASN map within 4.6 MB and could be commonly affected by genomic events leading to increased copy number in breast and other cancers
Experimental evidence has begun to support the concept that increased copy number at the ERBB2 amplicon allows cancer cells to produce high levels of intracellular lipid, while concomitantly promoting the conversion of FFA to triglycerides to avoid lipotoxicity [61, 62]. It has been proposed that the co-amplification of other lipogenic genes with ERBB2 further increases the reliance of such tumors on lipogenesis [62]. Two genes that have been identified to be important for ERBB2-positive breast cancer cell survival, but not that of other breast cancer cells or normal mammary epithelial cells, are mediator complex subunit 1 (MED1, previously known as peroxisome proliferator-activated receptor (PPAR) γ-binding protein or PBP) and the nuclear receptor NR1D1 (nuclear receptor subfamily 1, group D, member 1), a PPARγ target protein [62]. The MED1 and NR1D1 genes within the ERBB2 amplicon (see Fig. 11.4) not only positively affect transcriptional rates of the lipogenic genes FASN, ACLY, and ACACA but also further regulate lipid storage during adipocyte differentiation [63–68]. More recent experimental evidence supports the notion that co-amplification of MED1 and NR1D1 synergistically enhances FFA to triglyceride conversion in ERBB2-positive cells in order to avoid lipotoxicity [39, 62].
Lipogenic amplification target genes have also been identified at other genomic loci beyond chromosome 17q. For example, the “Spot 14” (S14 or THRSP) gene encodes a nuclear protein that is associated with fatty acid synthesis and is located at chromosome 11q13 [69]. High Spot 14 levels as detected by immunohistochemistry were significantly associated with tumor recurrence in breast cancer, but were not associated with either hormone receptor or ERBB2 status in the cohort examined [70].
Overweight and Obesity as Causes of Breast Cancer
Since the 1980s, the percentages of overweight and obese adults and children have risen markedly in the Western world, leading to an impending global health crisis of unprecedented proportion. This has been attributed to a combination of ready access to calorie-rich foods and reduced rates of activity. Overweight and obesity also represent a major environmental cause of cancer [71], which may overtake tobacco use as the leading such cause of cancer as smoking rates decline. The manner in which obesity predisposes individuals to cancer is still a subject of debate, and the causal role that obesity plays is likely to be different in the case of different cancer types. It may be difficult to separate, for example, the effects of obesity from the effects of lack of exercise, or from increased or reduced intakes of particular dietary components, which may have effects beyond contributing to the overweight or obese state.
Increased circulating levels of estrogen serve to drive the proliferation of estrogen receptor-positive breast cancers, and to date, the significance of obesity in relation to breast cancer incidence and risk has been proposed to lie primarily in adipose tissue representing the major site for estrogen synthesis in postmenopausal women [72]. In this tissue, estrogen is synthesized from androgens by aromatase, which is a major drug target in postmenopausal women with estrogen receptor-positive disease. Women with large breasts were reported to have a higher incidence of breast cancer relative to women with average-sized breasts, which could reflect amounts of increased glandular tissue from which tumors can derive and/or higher local estrogen levels generated from increased adipose tissue [73]. Obesity is also known to result in adipose tissue becoming increasingly dysfunctional, leading to the secretion of a variety of factors termed adipokines, which may promote tumor initiation or progression [74]. However, it is possible that a high-fat diet leading to obesity may also promote breast cancer through other mechanisms, as will be discussed below.
Diet and Breast Cancer
A large body of evidence substantiates an important role for de novo lipogenesis in cancer. Given the fact that breast cancer derives from cells with the ability to both synthesize lipid and take up lipid from the circulation, it is important to consider possibly dietary influences on breast cancer risk and development. To date, the role of dietary saturated fat in contributing to breast cancer risk is somewhat controversial. Positive associations between saturated fat or animal fat consumption and cancer have been reported in cohort studies [75] and in studies investigating cancer incidence in 20 countries [76]. Dietary intake of palmitic acid has also been significantly associated with increased breast cancer risk [77]. In general, inaccuracies in reporting dietary intake and difficulties in conducting mechanistic studies on human populations have hampered investigations on the role of dietary saturated fat in cancer development. However, measuring fatty acid composition of adipose tissue using lipidomics techniques may provide a composite measure of dietary fat intake over several years, due to the low turnover rate of stored lipids within adipose tissue [78].
Uptake of Dietary Lipids by Breast Cancer Cells: Part of the Picture?
While there are difficulties in conducting epidemiological dietary studies, some molecular studies have indicated the possible involvement of fatty acid uptake (in particular, saturated fatty acid uptake) in fuelling cancer cells. Studies have shown that LDL receptors are upregulated in tumor cells [79]; therefore, the LDL receptor-mediated pathway is a possible route for fatty acid delivery to peripheral tissues, especially tumor cells. Kuemmerle et al. also reported that cancer cells could also uptake released fatty acid from the lipolysis process through fatty acid translocase CD36 [80]. Immunohistochemical analysis confirmed the presence of LPL and CD36 in breast liposarcoma and prostate cancer tissues [80].
Excessive intake of dietary lipids is a well-known cause for obesity, which is in turn a risk factor for breast cancer [81, 82]. In the mammary gland, a large percentage of the cells are adipocyte or adipocyte precursor cells [83]. The abdominal fatty tissue known as the omentum has been described as a preferred metastasis location for ovarian cancer. Nieman et al. reported that adipocyte-ovarian cancer coculture led to the direct transfer of lipids from adipocytes to ovarian cancer cells and promoted in vitro and in vivo tumor growth [84]. Furthermore, coculture induced lipolysis in adipocytes and β-oxidation in cancer cells, suggesting that lipids stored in adipocytes can act as an energy source for the cancer cells [84]. Considering that the breast is an organ rich in adipose tissue, the transfer of fatty acids between breast cancer cells and breast adipocytes could also occur.
Therapeutic Targeting of Lipogenesis in Breast Cancer
A number of approaches have either been tested or may be applied to target lipogenesis in breast cancer. However, despite overwhelming evidence of the importance of lipogenesis in cancer, and in breast cancer in particular, progress in targeting this pathway has been described as modest at best [85]. Limiting factors have been described as the previous lack of crystallographic structures for relevant targets that has impeded drug design and in establishing structure–antitumor relationships [85]. The most heavily investigated target to date is FASN. Numerous FASN inhibitors have been reported and tested in the context of breast cancer, but their application has been limited in some cases by anorexic side effects [86]. Researchers are continuing to develop alternative inhibitors without these side effects [87]. Other key metabolic enzymes that could represent therapeutic targets in cancer cells are ACACA and ACLY. These targets are of great interest for the treatment of diabetes and obesity but have been explored to a limited extent in the context of cancer [85].
Due to the health and economic impact of the obesity pandemic, novel therapies are being aggressively developed against a variety of targets [88]. With rapidly improving knowledge of the significance of altered lipid synthesis and possibly uptake by cancer cells, such agents show increasing possibility of being adopted for cancer use. Redeploying approved drugs has advantages over the development of novel agents, in that there are preexisting pharmacokinetic, toxicity, and side effects data. For example, agents targeting fatty acid-binding proteins are being developed in the context of insulin resistance and other conditions [89] but could conceptually be applied to cancers where fatty acid-binding proteins are known to be overexpressed. The PAT protein family, which regulates lipid storage in lipid droplets, is also viewed as potential drug targets in the treatment of obesity [88] and are expressed in some lipogenic cancers [90]. The eventual targeting of lipid droplet-associated proteins could be applied to treat lipogenic cancers characterized by increased expression of these targets, where the overexpression of lipogenic genes may represent predictive biomarkers.
Characterizing Lipogenesis in Breast Cancer Cells
Lipid Detection Methods
Thin-layer chromatography (TLC), gas chromatography (GC), and high-performance liquid chromatography (HPLC) have been used in lipid research for many years. In 1966, Rees et al. studied the influence of hormonal status on lipid composition of rat mammary carcinomas, mammary glands, and related tissue [21]. In this study, they used TLC and GC to identify the levels of glycerolipids, sterols, and phospholipids relative to the percentage of total lipids. Following its emergence, TLC became widely accepted as a conventional analysis method for lipids in the 1960s [91, 92], with the advantages of being fast, simple, and inexpensive. However, the major limitation of TLC is its restricted resolution, which significantly hinders its application.
Since most lipids are not volatile and some lipids are easily degraded under high temperature, GC is not a very widely used method in lipidomics, due to the complexity of derivatization required before separation [93]. The derivatization may eliminate much structural information about lipid molecular species, especially polar lipids. Therefore, when using GC to analyze different categories of lipids, complex pre-separation is absolutely necessary [94]. These problems result in the much less frequent application of GC than liquid chromatography. Nevertheless, GC technology is appropriate for the analysis of fatty acids, because the resolution capacity of GC is much higher than that of liquid chromatography. The separation of cis/trans isomers, which is rarely achieved with other lipid detection methods, can be achieved using conventional GC–MS methods.
HPLC is the most widely used separation technique in lipidomics. In contrast to other separation techniques, HPLC has good reproducibility and high resolution and can separate almost all lipid molecular species. HPLC systems are relatively isolated from the environment, limiting the contact between samples and air and thus avoiding self-oxidation and degradation of lipids. In recent years, lipid separation by liquid chromatography and detection by mass spectrometry has become one of the core techniques for the growing field of lipidomics (see the section “Lipidomic Approaches”).
Other methods to detect lipids in biological systems include nuclear magnetic resonance (NMR) spectroscopy and biochemical approaches. NMR spectroscopy is an excellent tool to study molecular structures of purified lipids (1H-NMR and 13C-NMR) and for investigating the structure and dynamics of lipid membranes (1H, 2H, and 31P high-resolution and solid-state NMR) [95]. For the analysis of phospholipid mixtures, 31P-NMR is by far the most appropriate approach. The linear response and relatively high speed of 31P-NMR allow for accurate and selective analysis with high sample throughput [96]. One disadvantage is that NMR techniques have only moderate sensitivity compared with mass spectrometry. Many lipids can also be detected using biochemical approaches (e.g., optical/colorimetric assays). This type of measurement is highly quantitative, but often experimentally challenging in that optimization of conditions can require significant effort.
Lipidomic Approaches
The term “lipidome” describes the complete lipid profile within a cell, tissue, or organism and is a subset of the “metabolome,” which also includes the three other major classes of biological molecules, namely amino acids, sugars, and nucleic acids [97]. Efforts to characterize lipids in cells are relatively recent and have been driven by some spectacular advances in mass spectrometry instrumentation and applications. The dramatic increase in lipidomic research over the past decade has been triggered by impressive developments in analytical technologies, initiated by the application of electrospray ionization mass spectrometry (ESI-MS) to the characterization of membrane phospholipids [98–101]. Technical developments include very high sensitivity and specificity mass and chromatographic resolutions and the increased availability of authentic synthetic lipid standards. These, coupled with impressive developments in data and bioinformatics analysis, have facilitated the detailed molecular analysis of a wide diversity of lipids, ranging from phospholipids and triglycerides to sterols and glycolipids.
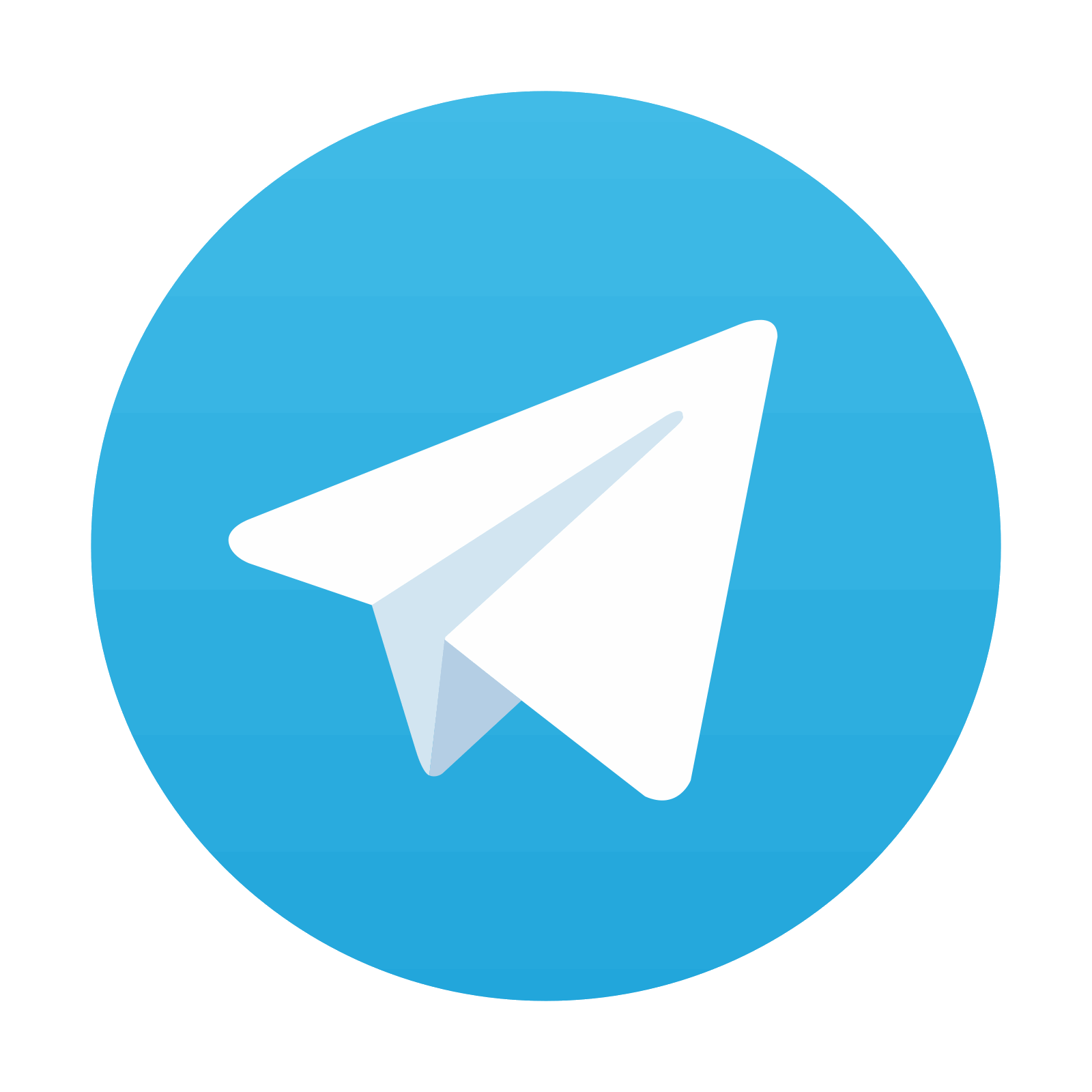
Stay updated, free articles. Join our Telegram channel
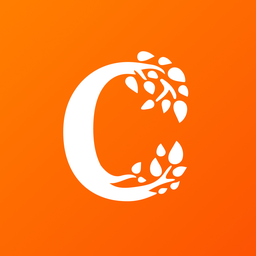
Full access? Get Clinical Tree
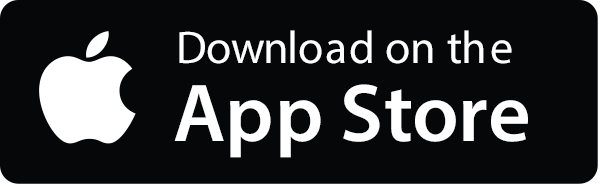
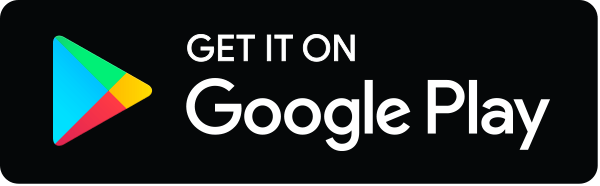