Keywords
Sodium iodide symporter (NIS), viral vector, lentivirus, radioiodide, tumor-specific targeting promoter
Sodium Iodide Symporter
Thyroid hormone synthesis requires a variety of molecules in thyroid follicular cells, including sodium iodide symporter (NIS), which transports iodide into the cells, and thyroperoxidase (TPO), which oxidizes iodide into iodinium (I + ) that in turn iodinates thyroglobulin (Tg) ( Figure 32.1 ) . The process of iodide transport and thyroid hormone synthesis leads the thyroid gland to concentrate iodide by a factor of 20–40 compared to the plasma level . The rat and human NIS (rNIS and hNIS, respectively) cDNA were cloned in 1996 , and murine NIS (mNIS) cDNA was reported in 2001 . These three NIS share highly conserved homology in cDNA and amino sequence (from 84 to 95%). NIS is a highly specialized membrane glycoprotein that possesses 13 transmembrane domains composed of 643 (hNIS) or 618 (rNIS and mNIS) amino acids, with its carboxyl end in the cytoplasm and amino end on the extracellular surface . It expresses mainly in the basolateral membrane of thyroid follicular cells, although less expression can also be found in several nonthyroidal tissues, such as salivary glands, mammary glands during lactation, kidney, and ovaries . NIS gene expression in thyroid follicular cells is tightly regulated by the thyrotropin cAMP pathway, which is crucial in maintaining the thyroid function in normal physiological condition . Upon binding of thyroid-stimulating hormone (TSH), activated TSH receptor (TSHR) dissociates heterotrimeric G protein, and the released Gα s subunit in turn activates adenylyl cyclase, followed by cAMP accumulation in the cells . This pathway regulates the proliferation, function, and differentiation of thyroid cells . Distinct regulatory elements in the 5′ flanking region and their corresponding transcription factors of NIS gene are involved in the TSH-related transcriptional regulation. The binding site of thyroid transcription factor 1 (TTF-1) was identified to be located between −245 and −230 bp in the rNIS 5′ flanking region . TSH-responsive element (TRE), the binding site of NIS TSH responsive factor 1 (NTF-1), is between –420 and –385 bp upstream from the TTF-1 binding site . In addition, NIS upstream enhancer (NUE) located between −2495 and −2264 bp was disclosed to regulate NIS transcription in the cAMP manner, and two binding sites of Pax8 transcription factor were found located in the NUE .

Radioisotopes for Nis Gene Expression Imaging and Therapy
Driven by the electrochemical sodium gradient generated by the Na + /K + -ATPase, NIS actively transports two sodium ions together with one iodide into thyroid follicular cells under normal physiological condition ( Figure 32.1 ) . Nevertheless, several substrates are able to be taken up by NIS. Coupled with two sodium, NIS is able to transport anions such as ClO 3 − , SCN − , SeCN − , NO 3 − , Br − , BF 4 − , IO 4 − , and BrO 3 − . NIS also transports technetium pertechnetate (TcO 4 − ), rhenium perrhenate (ReO 4 − ), and perchlorate (ClO 4 − ) . Because of the ability to transport a variety of substrates, radioactive pharmaceuticals derived from these substrates, such as technetium-99m ( 99m Tc), 123 I, 124 I, 125 I, 131 I, 188 Re, and 211 At, can be used in NIS-mediated imaging and/or therapy. These radiopharmaceuticals have the advantage that they can be directly used without further labeling in NIS-mediated gene imaging and therapy. Radioiodide has been successfully used to treat hyperthyroidism from Graves’ disease and metastatic thyroid cancer . 131 I is a β and γ emitter with a half-life of 8 days. The major emitted β particles have a maximal energy of 606 keV (89% abundance; others, 248–807 keV), and those of γ rays have a maximal energy of 364 keV (81% abundance) . Because it emits both β and γ rays, 131 I can be used in NIS-mediated imaging and therapy. However, its high energy of emitted γ rays results in poor image quality; therefore, 131 I is not recommended for imaging. 123 I has a half-life of 13.2 hr. As it decays by electron capture, 123 I emits γ rays with predominant energies of 159 and 127 keV. The 159-keV γ rays are ideal for detection by the NaI (sodium iodide) crystal detector of current gamma cameras. 123 I also has a counting rate 20-fold higher than that of 131 I and therefore provides a higher lesion-to-background signal and better image quality. Moreover, 123 I delivers a relatively lower absorbed radiation dose, approximately one-fifth of 131 I . 124 I, with a half-life of 4.2 days, is a positron emitter used for positron emission tomography (PET), and it may offer the best image quality with better efficiency and resolution . 125 I is a β and γ emitter with a half-life of 59.4 days. It initially decays by electron capture to tellurium-125, which immediately decays with emitting 35 keV γ rays. During the decay process, the excess energy is internally converted into 21 Auger electrons with very low energies (50–500 eV) . For NIS-based imaging, 123 I is preferred compared to 125 I due to better tissue penetration and shorter half-life. For NIS-based therapy, 131 I provides more effective cell killing than 125 I. Nevertheless, 125 I is extensively used in in vitro iodide cellular uptake to examine the expression and function of NIS. 99m Tc is a metastable nuclear isomer of Tc-99 and is the most commonly used radioisotope in nuclear medicine. It has a short half-life of 6 hr and emits 140 keV γ rays. The short physical and biological half-life (6 hr and 1 day, respectively) of 99m Tc enable rapid data collection and low radiation exposure of patients. 99m Tc pertechnetate is useful for NIS or thyroid studies without further labeling to thyroid-specific compounds . 211 At is the heaviest α-emitting radiohalide, with a half-life of 7.2 hr. The α particles emitted with a mean energy of 6.7 MeV (42% 5.9 MeV and 58% 7.5 MeV) cause a 60-mm tissue penetration and remarkably high energy deposition. 211 At is currently an attractive and promising isotope for radiotherapy of cancer . 188 Re decays with a half-life of 17 hr and emits high-energy β particles (maximal energy of 2.12 MeV) and γ rays (15% 155 keV) and thus is suitable for imaging as well as cancer therapy. Due to the higher energy of β rays and a shorter half-life, 188 Re may deposit higher energy than 131 I in NIS-expressing tumors during a short period of time .
Multimodality Imaging for Nis Gene Expression
Monitoring and quantifying the transgene expression in vivo is an important issue in gene therapy. Expression of a transgene measured by an effective imaging system will provide useful information of curative effect before gene therapy is carried out. An ideal in vivo imaging system should allow transgene expression to be monitored over time with regard to the location, magnitude, and kinetics . Due to the unique capacity of transporting various radionuclides, including α, β, and γ emitters, NIS can be used as both a therapeutic gene and a reporter gene. Several imaging modalities, including gamma camera, PET, single-photon emission computed tomography (SPECT), and luminescence imaging for Cerenkov radiation, can be used to monitor in vivo NIS gene expression ( Figure 32.2 ). By detection of temporal and spatial change of radioisotope accumulation and metabolism in the living subject, these modalities can precisely monitor the expression level of NIS as well as evaluate the therapeutic outcome. Due to rapid advancements, nuclear imaging modalities designed for small animal study have achieved a spatial resolution of less than 2 mm. Combined modalities are also being developed, including SPECT/CT, PET/CT, PET/SPECT/CT, and PET/magnetic resonance imaging, which generate fused images comprising both functional and anatomical information . A recent study combined Cerenkov luminescence and nuclear imaging for detection of NIS gene expression. Injection with 124 I enabled the thyroid gland or NIS-expressing tumor to be imaged with both PET and optical imaging equipment. This technology has broadened the application of NIS for future diagnostic development . Although with lower production of Cerenkov luminescence, 131 I is still a potential radiopharmaceutical used for optical imaging to evaluate thyroid function and monitor the treatment of thyroid cancer .

Nis-Mediated Cancer Gene Therapy
Gene therapy is commonly designed for treatment of disease by correcting a mutated gene in an inherited disease. It is completed by introducing a DNA sequence encoding a functional protein in order to replace the mutated gene. Cancer gene therapy requires a transgene with its encoded product that can induce cell killing effect. These include genes encoding pro-apoptotic proteins p53, E1A, p202, PEA3, BAX, and Bik or anti-angiogenic proteins such as angiostatin, endostatin, interleukin-12, and interferon-α . Another promising approach is cancer suicide gene therapy. The most documented examples are prodrug-metabolizing enzymes such as herpes simplex virus thymidine kinase (HSV-TK) and cytosine deaminase (CD). HSV-TK phosphorylates nucleoside analogs such as ganciclovir and acyclovir, which are normally not phosphorylated in eukaryotic cells. The incorporation of these phosphorylated analogs into the replicating DNA terminates the DNA replication, resulting in cell death. In several bacteria or fungi, CD deaminates cytosine and subsequently turns into uracil. This reaction is applied in suicide gene therapy with the introduction of the CD gene into cancer cells followed by administration of the relatively nontoxic prodrug 5-fluorocytosine (5-FC). 5-FC is deaminated by CD to the highly toxic drug 5-fluorouracil (5-FU) and leads to cell death by interfering with RNA processing and DNA synthesis . Use of NIS for suicide cancer gene therapy follows a different mechanism that mediates the transport of 131 I into cells and results in cell killing by β particles ( Figure 32.3 ). Shimura et al . first reported stable transfection of rNIS into malignant transformed rat thyroid cells (FRTL-Tc) and showed a 60-fold increase in 125 I accumulation in vitro . However, in the attempt to use in vivo 131 I therapy, no significant tumor volume reduction was observed, which might be attributed to the short effective half-life of 131 I accumulated in the tumor. Mandell et al . reported the selective killing effect of 131 I on various rNIS-transduced cancers, such as melanoma, liver cancer, colon carcinoma, and ovarian carcinoma cell lines. Many robust studies have demonstrated the feasibility of NIS-mediated gene therapy for various kinds of cancer ( Table 32.1 ). The use of 188 Re and 211 At in NIS-mediated cancer gene therapy has also been reported. Both 188 Re and 211 At potentially exhibited a more effective therapeutic effect than 131 I .

Year | Authors | Cell Type | NIS | Promoter | Vector | Radionuclide |
---|---|---|---|---|---|---|
2000 | Spitzweg et al . | LNCap (prostate cancer) | Human | PSA | Stable transfection (liposome) | 131 I |
2001 | Spitzweg et al . | LNCap (prostate cancer) | Human | CMV | Adenovirus | 131 I |
2002 | Smit et al . | FTC133 (thyroid cancer) | Human | CMV | Stable transfection (lipid) | 131 I |
2004 | Shen et al . | F98 and RG2 (gliomas) | Human | CMV | Retrovirus | 131 I/ 125 I/ 188 Re |
2004 | Faivre et al . | HCC (chemical induced) | Rat | CMV | Adenovirus | 131 I |
2004 | Mitrofanova et al . | DU145 (prostate cancer) | Rat | CMV | Adenovirus | 131 I |
2004 | Gaut et al . | FaDu (HNSCC) | Human | CMV | Adenovirus | 131 I |
2005 | Dwyer et al . | T47D (breast cancer) | Human | MUC1 | Adenovirus | 131 I |
2006 | Dwyer et al . | OVCAR-3 (ovarian cancer) | Human | MUC1 | Adenovirus | 131 I |
2006 | Dwyer et al . | Capan-2 (pancreatic cancer) | Human | MUC/CMV | Adenovirus | 131 I |
2006 | Chen et al . | MH3924A (hepatoma) | Human | mAlb | Retrovirus | 131 I |
2006 | Petrich et al . | K1 (thyroid cancer) | Human | CMV | Stable transfection | 211 At |
2006 | Mitrofanova et al . | DU145 (prostate cancer) | Rat | CMV | Adenovirus | 131 I |
2007 | Schipper et al . | Bon1 (neuroendocrine cancer) | Human | CMV/CgA | Stable transfection (electroporation) | 131 I/ 99m Tc |
2007 | Spitzweg et al . | TT (thyroid cancer) | Human | CEA | Adenovirus | 131 I |
2007 | Willhauck et al . | LNCap (prostate cancer) | Human | PSA | Stable transfection (liposome) | 188 Re/ 131 I |
2007 | Kim et al . | CT26 (colon cancer) | Human | UbC | Lentivirus | 131 I |
2007 | Chen et al . | Capan-2 (pancreatic cancer) | Human | MUC1 | Adenovirus | 131 I |
2007 | Hsieh et al . | ARO (thyroid cancer) | Human | CMV | Stable transfection (polyethylenimine) | 131 I |
2008 | Kim et al . | Hep3B (hepatoma) | Human | hTERT | Retrovirus | 131 I |
2008 | Montiel-Equihua et al . | ZR 75-1 (breast cancer) | Human | CMV/ERE | Adenovirus | 131 I |
2008 | Willhauck et al . | HepG2 (hepatoma) | Human | AFP | Stable transfection (liposome) | 131 I |
2008 | Willhauck et al . | LNCap (prostate cancer) | Human | PSA | Stable transfection (liposome) | 211 At |
2008 | Jin et al . | Huh-7 (hepatoma) | Human | AFP | Stable transfection (liposome) | 131 I |
2009 | Msaouel et al . | LNCaP (prostate cancer) | Human | CMV | Measles virus | 131 I |
2010 | Ahn et al . | HCT-15 (colon cancer) | Human | CMV | Adenovirus | 131 I |
2010 | Lee et al . | ARO (thyroid cancer) | Rat | CMV | Stable transfection (liposome) | 188 Re |
2011 | Huang et al. | PC-3 (prostate cancer) | Human | survivin | Adenovirus | 131 I |
2011 | Riesco-Eizaguirre et al . | M14 (melanoma) | Human | hTERT/CMV | Adenovirus | 131 I |
2011 | Klutz et al . | HepG2 (hepatoma) | Human | mAFP | Adenovirus | 188 Re/ 131 I |
2011 | Ke et al . | ARO (thyroid cancer) | Human | CMV | Lentivirus | 131 I |
Nis Gene Delivery
Delivery of a therapeutic gene into target cells is the initial step of gene therapy. The efficiency of gene delivery is one of the major determining factors of therapeutic outcome. Nonviral and viral methods have been applied to deliver DNA into cells.
Nonviral Methods
To ectopically express NIS in cancer cells with no or reduced NIS expression, several nonviral methods have been used to deliver NIS. Electroporation increases the cell permeability by giving an electric field to cells, resulting in the enhancement of plasmid DNA uptake into cells. Stable cell clones expressing NIS have been established by using this method . Lipid-based carrier such as liposome is the mainstay in gene transfer. This positively charged, lipid bilayer component forms a complex with negatively charged DNA and interacts with the negatively charged cell membrane, facilitating contact and delivery of the DNA to the cell. This is the most commonly used nonviral method to transfer NIS in cancer cells . Polymer-based carrier such as polyethylenimine (PEI) is also a well-developed agent for DNA delivery . PEI condenses DNA into positively charged particles, which interact with anionic proteoglycans on the cell surface, resulting in endocytosis. The polymer–DNA complex is eventually released in the cytoplasm due to osmotic swelling and rupture of endosome. Using this approach, we successfully transfected the anaplastic thyroid cancer cell line, ARO, with the NIS gene . The major disadvantages of nonviral methods are the low transfection efficiency and transient expression of transgene, which hampers their use in in vivo NIS delivery. Currently, the nonviral methods are mainly used to generate stable cells by antibiotic selection. For this purpose, a selection marker on either the same construct or a separated one is cotransfected with transgene into cells. To generate cells stably expressing the transgene after transfection, integration of transgene as well as selection marker into chromosomal DNA in the host cells is required, although it is a rare event. Antibiotic selection first screens the cells that express selection marker, and the surviving cells are then confirmed for the the transgene expression. Evaluation of the expression of mRNA by reverse-transcription polymerase chain reaction and protein by Western blot provides direct evidence of NIS expression in the selected transfected cells. NIS function can be assessed by in vitro radioiodide uptake.
Viral Methods
Viruses interact with the host cell surface and transfer their genetic material into the cells, where the viral genes are transcribed as part of the viral replication cycle. In gene therapy, all or a portion of the viral genes are replaced with the therapeutic gene. Viruses are divided into two classes depending on their replication cycle—the lytic and nonlytic viruses. Nonlytic viruses, such as retroviruses and lentiviruses, infect and exploit the machinery of cells without killing them. In contrast, infection by lytic viruses, such as herpes simplex viruses and Semliki Forest viruses, leads to eventual cell death.
Adenoviral Vector
The most commonly used NIS gene transfer vehicle for imaging and therapy is the adenoviral vector, which has the advantages of the ability to infect both dividing and nondividing cells, easy manipulation, and high titer production. Adenoviruses are nonenveloped, double-stranded DNA virus enclosed by an icosahedral capsid . Infection of adenovirus begins with the binding of the viral fiber knob to the coxsackie virus–adenovirus receptor of target cells. The interaction of viral capsid penton base Arg-Gly-Asp (RGD) motif and cellular α v β integrin leads to internalization of virus, which initializes the endocytosis . After the virus escapes from endosome, viral DNA translocates to the nucleus and is expressed. The viral genome encodes two groups of transcriptional units, early and late, according to their transcription occurring in the early and late phases. Events in the early and late phases occur before and after initiation of viral DNA replication, respectively. In the early phase, four noncontiguous regions encode six distinct regulatory proteins—E1a, E1b, E2a, E2b, E3, and E4—that act in the infected cells and halt protein synthesis, inhibit p53 function, avoid premature death of host cells, and activate other viral genes encoding proteins for the use of subsequent viral DNA replication . Viral DNA replication, production of structural proteins, and viral particle packaging occur in the late phase. Eventually, viruses are released as a result of cell lysis. For gene therapy, nonreplicative adenoviral vectors were generated to meet safety concerns. First-generation adenoviral vectors containing the whole viral genome but lacking the E1 region were developed . These adenoviruses can be prepared and propagated in the E1-expressing cell line . E1-deleted adenovirus can efficiently transduce most cells but is defective in replication after infection. However, proteins encoded by the remaining genes still induce the immune response in vivo toward transduced cells, which eventually are eliminated, resulting in a reduced period of transgene expression and loss of the therapeutic effect. To overcome this problem, second-generation adenoviral vectors that may carry additional deletions of E2 and E4 regions and result in a 14-kb capacity for gene cloning are derived . However, this generation of viruses still does not avoid the problem of immune response in vivo . Third-generation vectors, with removal of all E regions, are developed as the “gutless” adenoviral vector, which keeps only the 5′ and 3′ inverted terminal repeats and the packaging signal, and leaves up to 36-kb capacity for DNA cloning . Adenoviral infection does not lead to viral DNA integration to host genome; therefore, there is no concern for genetic alteration. Nevertheless, the nonintegrating property results in the transient expression of adenoviral gene transfer and therefore limits the efficacy in gene therapy .
Replication-defective adenoviral vector encoding hNIS and rNIS genes was constructed in 2000 by Cho et al ., who showed in vitro and in vivo hNIS gene delivery by adenovirus to glioma cells/tumors resulting in successful NIS expression and radioiodide uptake . Boland et al . demonstrated the infection of cell lines derived from cervical, breast, prostate, lung, and colon cancers with adenovirus carrying rNIS . The infected cells showed a 125- to 255-fold-increase in 125 I uptake in vitro compared to parental cells. In vivo imaging 3 days after intratumoral injection of adenovirus showed that the tumors accumulated 4- to 25-fold more 125 I or 123 I compared to noninfected tumors . These results indicated the promising potential of adenoviral NIS transfer for radionuclide tumor therapy. Spitzweg et al . used tumors derived from prostate cancer cells as a model for adenoviral NIS transduction followed by 131 I therapy . Following intraperitoneal administration of 131 I, the tumors transduced with adenovirus encoding NIS showed an average volume reduction of 84±12%. This pioneering study paved the way for therapeutic application of NIS gene delivery followed by radioiodine therapy in prostate cancer patients in a clinical setting. Several reports on the use of adenoviral-mediated NIS transfer into different cancer cell lines derived from prostate, breast, liver, colon, ovary, and thyroid cancers have been published . All data indicated that NIS can be efficiently expressed and can actively transport iodide in the transfected cells. Another study using adenovirus encoding rNIS and 3 mCi of 131 I reported an immediate inhibition of growth in 131 I-treated tumors . A lower therapeutic dose of 131 I of 1 mCi in the same model also achieved a comparable inhibition of tumor growth . The same approach was effectively applied to other tumor models, including head and neck cancer , breast cancer , ovarian cancer , and pancreatic cancer . Due to their easy manipulation, adenoviral vectors are widely used in NIS gene transfer. However, the problems of transient gene expression and adverse immune response in in vivo use remain to be overcome.
Retroviral Vector
Retroviruses are enveloped single-stranded RNA viruses that belong to the large Retroviridae family. The virions are approximately 100 nm in diameter and spherical, and the outer layer is a lipid envelope displaying viral glycoproteins. A mature retrovirus contains three major components: the envelope, the genomic RNA, and the proteins. The single-strand RNA genome contains two long terminal repeat (LTR) sequences at both ends that frame three major genes—gag, pol, and env, which encode the structural proteins, nucleic acid polymerases/integrases, and surface glycoprotein, respectively. LTRs are important for the initiation of viral DNA synthesis, integration, and regulation of viral transcription . The envelope is composed of glycoproteins encoded by the env gene and lipids obtained from the host plasma membrane. Infection begins with the binding of the surface envelope glycoprotein of the virus to a receptor on the surface of the host cell. This interaction leads to the fusion of the viral and cellular membrane, followed by release of the viral RNA and proteins in the host cytoplasm. The single-strand RNA genome of the virus is converted into double-strand linear DNA by the enzyme reverse transcriptase. The double-strand viral DNA is then transported from the cytoplasm to the nucleus and integrates into the host genome, termed provirus. Evidence indicates the existence of preferential sites of retroviral integration . Integration of retroviral DNA raises the risk of mutagenesis of host cells. Increased, decreased, or even disrupted expression of genes may occur when viral DNA integrates into the adjacent site of these genes. Silencing of a tumor suppressor gene or activation of oncogene in the host cell following viral DNA integration can lead to oncogenesis. Thus, considerable attention needs to be paid to the safety of the retroviral transfection, particularly the possibility of formation of replication-competent viruses through recombination. Three or four plasmid production systems with minimal sequence homology have thus been developed . The expression of the integrated provirus is also influenced by the site of integration. A replication-defective retroviral system for ectopic gene delivery can be generated by separating the provirus sequences into the transfer vector and the packaging vector. In addition to the gene of interest, the transfer vector retains the LTR region, primer binding site, and RNA packaging signal required for viral integration, replication, and reverse transcription. The gag/pol and env sequences are cloned in the packaging vector. The produced viruses carry genomic RNA with no sequence-encoding viral proteins, and the resulting provirus in the host cells no longer undergoes replication.
Mandell et al . successfully demonstrated the rat NIS-mediated iodide uptake after retroviral transduction of human melanoma cells, mouse transformed liver, mouse colon carcinoma, and human ovarian adenocarcinoma . These transduced cells showed effective 131 I cell killing, and the rNIS-transduced tumors can be visualized by radionuclide imaging after 123 I administration. Retroviral transfer of the human NIS gene and the hygromycin-resistance gene to Morris hepatoma cells followed by hygromycin selection generated several hNIS expression cell lines . All cell lines showed significant iodide uptake even though the random integration of retrovirus resulted in various levels of iodide accumulation among individual cell lines. After treatment of sodium perchlorate, iodide uptake was decreased in a dose-dependent manner, indicating that hNIS expression contributes to iodide accumulation. With the promising feature of iodide uptake by NIS, the location and/or therapeutic effect of NIS-transduced subcutaneous prostate tumor, metastatic prostate tumor . and intracerebral glioma can be monitored by this imaging approach.
Lentiviral Vector
Lentivirus, which is one type of retrovirus, also belongs to the Retroviridae family and is structurally similar to retroviruses but has a more complex genome. In addition to the gag, pol, and env genes, two essential regulatory genes—tat and rev—as well as other accessory genes comprise the lentiviral genome. The tat gene encodes the Tat transactivator required to promote expression of full-length vector RNA. The rev gene encodes the Rev protein required for nucleocytoplasmic transport of full-length vector RNA and packaging RNAs. Other sequences include Rev response element, which is bound by Rev to mediate nucleocytoplasmic transport and pA, a polyadenylation signal. Unlike retrovirus, which infects only dividing cells, lentivirus can infect both dividing and nondividing cells because their preintegration complex (PIC) can penetrate the intact membrane of the nucleus of the target cell. After internalization of the virus into the target cell, the retroviral genome is contained in a PIC, which is actively transported to the nucleus of the target cell by a process that requires ATP but is independent of cell division . This process enables lentivirus to transduce nondividing or terminally differentiated cells, such as lymphocytes, dendritic cells, neurons, macrophages, hematopoietic stem cells, retinal photoreceptors, and muscle and liver cells, to which the previous gene therapy methods could not be successfully applied . Quiescent cells in the G 0 state are the only cells that cannot be infected successfully by lentiviruses because it blocks the reverse transcription step . Human immunodeficiency virus type 1 (HIV-1), which causes AIDS, is a typical lentivirus and remains the most studied lentiviral vector to date. Lentiviral vectors based on primate HIV-2 and simian immunodeficiency virus (SIV) are also being developed. In addition, nonprimate lentiviruses, such as feline immunodeficiency virus (FIV), equine infectious anemia virus (EIAV), caprine arthritis encephalitis virus (CAEV), and bovine immunodeficiency virus (BIV), have also been applied as gene therapy vectors .
Lentiviral-encoded NIS gene therapy was first conducted by Dingli et al. to target multiple myeloma . In patients with myeloma, the pool of actively replicating cells is usually less than 1%. Therefore, the lentivirus property of efficiently transducing nondividing cells is suitable and required to target the melanoma cells. Using immunoglobulin promoter and enhancer elements to specifically transfer NIS gene to myeloma cells, the lentiviral-transduced myeloma cells/tumor xenograft showed efficient radioiodide uptake. After 131 I treatment, the NIS-expressing xenograft regressed completely. Kim et al . transferred the NIS gene to a colon cancer line by lentiviral transduction . To establish a stable and long-term gene expression model, a ubiquitous promoter, human ubiquitin C, was used instead of cytomegalovirus (CMV), which was reported to have the problem of gene silencing when used in the in vivo gene therapy. The NIS-expressing tumor xenografts showed retarded growth after 131 I administration. We have demonstrated the use of lentiviral NIS expression controlled by a chimeric hepatitis B virus E2 enhancer and α-fetoprotein promoter to specifically target hepatoma cells. Compared to non-hepatoma cells, the transduced HepG2 cell line efficiently accumulates radioiodide, which was confirmed by 124 I microPET imaging . Recently, we transduced anaplastic thyroid cancer cells with the NIS gene by lentivirus, and an effective tumor growth inhibition was observed when NIS-expressing tumor xenografts were treated with 1.5 mCi of 131 I . However, when preparing the lentiviral particles, the problem of low titers was encountered, despite the fact that the titers can be increased by approximately 2 log after ultrahigh centrifugation. Compared to the adenovirus titer, this titer is not sufficient for in vivo studies . We also found that the susceptibility to lentiviral infection differs among different cell lines, resulting in different transduction efficiencies. Dingli et al . observed a wide range of transduction efficiency between 30 and 90% in myeloma and nonmyeloma cell lines infected by lentivirus . Pellinen et al . showed a wider range of 9–94% transduction efficiency among 42 cancer cell lines derived from bladder, bone, brain, breast, cervix, colon, lung, melanoma, pancreatic, and prostate cancers . Even different cell lines with the same tissue origin have major differences in gene transfer efficiency. For example, bone cancer cell lines MG-63 and U-2-OS have transduction efficiencies of 18.9 and 92.4%, respectively. In our study, we produced lentiviral vectors with titer of 10 8 to 10 9 VP/ml. Six successive infections led to a 5.8- and 17.7-fold radioiodide accumulation of transduced ARO and HepG2 cells, respectively, compared to their parental cells. The low transduction efficiency may be attributed to the low susceptibility of these two cell lines to lentiviral infection. Surprisingly, almost 3-fold higher iodide accumulation was observed in transduced HepG2 cells compared to ARO cells after lentiviral transduction following the same procedure. ARO is known as an anaplastic thyroid cancer, although the expression of several thyroid-specific genes, such as NIS, Tg, and TPO, is not detected. The low titer of lentivirus has limited in vivo transduction efficiency. After intratumoral injection of lentivirus in the tumor-bearing mouse model, no significant difference in radioiodide accumulation was observed between virus-injected tumors and parental tumors assessed by 124 I microPET imaging. The efficiency of lentiviral gene transfer needs to be improved by increasing the virus titer.
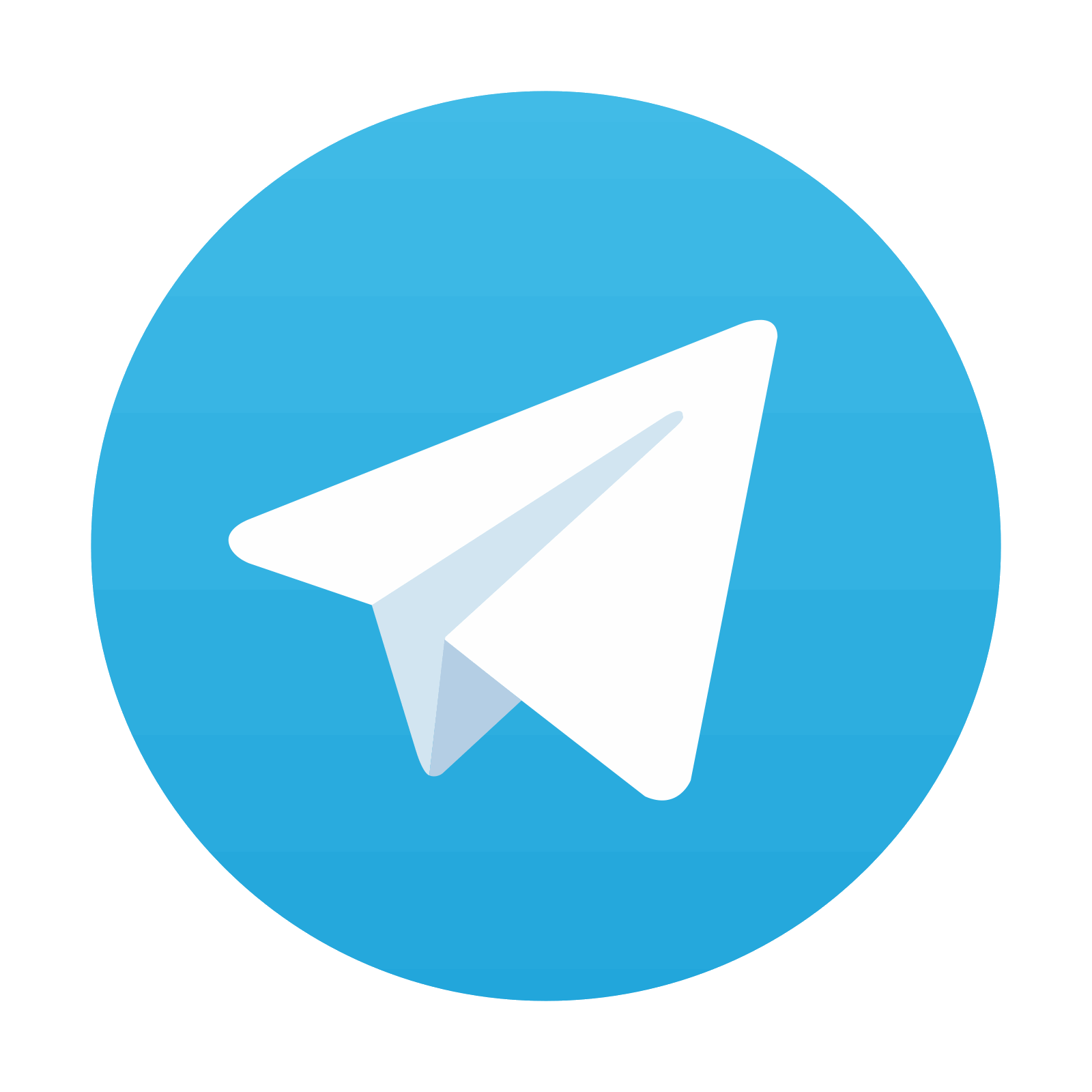
Stay updated, free articles. Join our Telegram channel
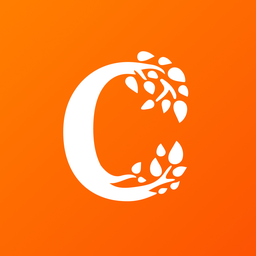
Full access? Get Clinical Tree
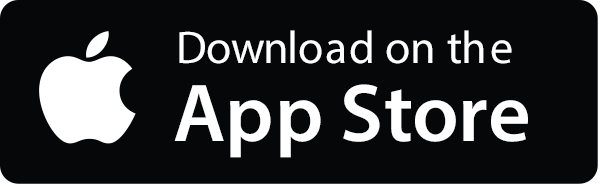
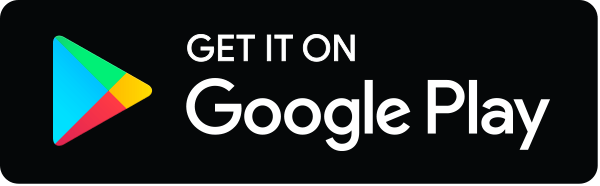