Chapter 2
Ion Channels and Electrical Activity in Pituitary Cells: A Modeling Perspective
Richard Bertram, Joël Tabak and Stanko S. Stojilkovic
Department of Mathematics and Program in Neuroscience, Florida State University, Tallahassee, FL, USA
Section on Cellular Signaling, National Institute of Child Health and Human Development, National Institutes of Health, Bethesda, MD, USA
2.1 Endocrine pituitary cells are electrically active
In 1975, Kidokoro showed that endocrine cells of the anterior pituitary were electrically excitable, producing action potentials like neurons do (Kidokoro, 1975). But why are pituitary cells excitable (Mollard and Schlegel, 1996)? Their role is to synthesize and secrete hormones in certain physiological conditions, with a time scale of minutes to hours (Stojilkovic et al., 2010). Thus, there is no need for fast action potentials that can transmit information on a millisecond time scale. Kidokoro and colleagues quickly realized that pituitary action potentials, which are wider than neuronal action potentials, are largely mediated by the influx of ions, with
ions playing a minor role (Kidokoro, 1975). Such action potentials act as
pulses to trigger
-dependent hormone secretion and other cellular processes (Stojilkovic, 2006).
The many types of ion channels present in the pituitary cell membranes regulate electrical excitability and determine whether a cell is silent, generates action potentials or bursts (Stojilkovic et al., 2010). Figure 2.1 shows several examples of spontaneous electrical activity in three types of endocrine pituitary cells. The recordings from somatotrophs and lactotrophs illustrate the fast bursting often seen in these cells. Occasionally, bursts consist of large spikes emanating from a depolarized plateau (top panel in Figure 2.1b). This is called plateau bursting. More often, the bursts have much smaller spikes (Figure 2.1a and lower three panels of Figure 2.1b). This is often called pseudo-plateau bursting (Stern et al., 2008; Teka et al., 2011). Gonadotrophs typically do not burst spontaneously, but instead produce action potentials with higher amplitude than those produced during bursting (Figure 2.1a). Although the gonadotroph action potentials are taller, they are also much narrower, so much less enters the cell with tonic action potentials than with bursts (Van Goor et al., 2001aa). This is clearly seen with measurements of intracellular
concentration (Figure 2.1). Electrical excitability provides a versatile way to control pituitary output: hypothalamic and pituitary factors as well as steroid hormones can modulate
influx in many ways by altering the electrical activity (Hodson et al., 2012; Stojilkovic, 2012).

Figure 2.1 Spontaneous activity in pituitary cells. (a) Simultaneous measurements of membrane potential () and the intracellular
concentration (
) in pituitary somatotrophs, lactotrophs, and gonadotrophs. (b) Left: Variations in patterns of spontaneous electrical activity and associated
transients in somatotrophs from the same preparation. Right: Expanded time scales, showing selected bursts labeled with asterisks on left.
2.2 Endocrine pituitary cell types
The pituitary gland is composed of two embryonically, anatomically, and functionally distinct entities: the neurohypophysis or posterior pituitary and the adenohypophysis, composed of intermediate and anterior pituitary lobes. The adenohypophysis is populated with three groups of secretory (endocrine) cell types: pro-opiomelanocortin-producing cells, growth hormone (GH)/prolactin-producing cells, and heterodimeric glucoprotein-producing cells. All pituitary cell types are modulated by gonadal steroid hormones and agonists for ligand receptor channels and G protein-coupled receptors in a cell-specific manner.
The pro-opiomelanocortin gene is highly expressed in melanotrophs and corticotrophs. Melanotrophs are located in the intermediate lobe where they represent about 95% of endocrine cells. Corticotrophs are derived from the intermediate pituitary, but in adult animals these cells are scattered throughout the anterior lobe and make up 10%–15% of endocrine anterior pituitary endocrine cells. Both cell types are electrically excitable, but spontaneous action potentials are sufficient to trigger the release of pro-opiomelanocortin-derived peptides only in melanotrophs. Melanotrophs are regulated through the actions of dopamine, -aminobutyric acid (GABA), prostaglandin E2 and 5-hydroxytryptamine (Vazquez-Martinez et al., 2003). Corticotrophs are primarily regulated through the action of corticotropin-releasing hormone, but also by urocortin 1–3 (a corticotropin-releasing hormone family of peptides) and arginine vasopressin (Aguilera et al., 2004).
Lactotrophs account for 10%–25% of the endocrine cells located in the anterior lobe. Spontaneous electrical activity in these cells is sufficient to trigger prolactin secretion. The predominant hypothalamic influence is inhibitory and is mediated by dopamine (Freeman et al., 2000). The sister cells, somatotrophs, represent up to 50% of all pituitary endocrine cells, and they are controlled by two hypothalamic neuropeptides: growth hormone-releasing hormone (GHRH), which stimulates GH release, and somatostatin, which inhibits GH release (DeAlmeida and Mayo, 2001; Patel et al., 1996).
Gonadotrophs and thyrotrophs express the 92 amino acid -glucoprotein hormone
-subunit, which is needed for the formation of follicle-stimulating hormone, luteinizing hormone, and thyroid-stimulating hormone with hormone-specific beta subunits. Gonadotrophs constitute about 10%–15% of the endocrine anterior pituitary cell population, while thyrotrophs constitute less than 10% of cells in the gland (Pazos-Moura et al., 2003). The decapeptide gonadotropin-releasing hormone is the main agonist for gonadotrophs and is secreted in a pulsatile manner by neurons that are dispersed within the mediobasal hypothalamus preoptic areas (Stojilkovic et al., 1994). Hypothalamic control of thyrotrophs is mediated by thyrotropin-releasing hormone, by numerous autocrine and paracrine factors.
2.3 Voltage-gated ion channels
Voltage-gated channels have been classified into two major subgroups: a superfamily of more than 140 members of voltage-gated cation channels that share structural similarity and a small family of structurally different voltage-gated chloride channels. Voltage-gated cation channels include ,
, and
channels, as well as numerous less selective channels. The majority of voltage-gated channels contain voltage sensors, charged transmembrane helices that sense the electrical field in the membrane and which drive conformation changes, leading to opening and closing of the gates near the mouth of the pore (Yu et al., 2005).
2.3.1 Voltage-gated sodium channels
Voltage-gated (
) channels are the primary molecules responsible for the generation of the rising phase of APs in excitable cells. The key electrophysiological features of these channels are: voltage-dependent activation, rapid inactivation, and selective
conductance. Tetrodotoxin (TTX) is a highly selective blocker of most types of voltage-gated
channels (Catterall et al., 2005). Pituitary cells express functional channels that are composed of TTX-sensitive and TTX-resistant components (Stojilkovic et al., 2012).
A mathematical model of the TTX-sensitive current is the product of a conductance and a driving force. The conductance has an activation factor and an inactivation factor. The current through that conductance is given by Ohm’s law as

where the Nernst potential, in our model, is such that the current is depolarizing. The maximal conductance
varies from cell to cell, since it is determined in part by the number of
channel proteins that are expressed in the plasma membrane. It is much larger in gonadotrophs than in lactotrophs and somatotrophs (Van Goor et al., 2001bb). The activation variable (
) and inactivation variable (
) change over time according to the differential equations


These equations come from the application of the law of mass action to a channel with independent activation and inactivation gates, each of which can be in an open or a closed state. The variable represents the fraction of channels with an open activation gate, and
is the fraction with an open inactivation gate. All voltage-dependent activation and inactivation variables satisfy equations of this form, and are thus said to satisfy first-order kinetics.
The steady-state activation and inactivation functions are Boltzmann functions, which have the form

The parameter sets the voltage at which the function is half-maximal (equal to ½). The parameter
sets the slope of the function; smaller values give the curve a more step-like appearance. The sign of
is typically positive for an activation function (increasing with voltage) and negative for an inactivation function (decreasing with voltage). The
and
parameters are called shape parameters since they control the shape of the activation/inactivation function. The
activation and inactivation functions are plotted in Figure 2.2. At the resting potential, the channel is deactivated (
), so the current does not contribute to the resting potential. In addition, the current is largely inactivated (
), so even if the cell becomes depolarized, the
current will not contribute much to the membrane potential. For lactotrophs and somatotrophs, where the maximal conductance
is also small, the current plays little role in the cell’s electrical activity.

Figure 2.2 Gating properties of channels. Steady-state activation function (
) and inactivation function (
) for the TTX-sensitive
current. Shape parameters are
,
,
, and
.
2.3.2 Voltage-gated calcium channels
Voltage-gated (
) channels are key sources of
entry into the cytosol in excitable cells. Based on their biophysical profiles,
channels are separated into two groups. The first group of channels is known as the high-voltage activated
channels, because these require moderate to strong membrane depolarization to open. Among this group, biophysical and pharmacological studies have identified the dihydropyridine-sensitive L-type (
1.1, 1.2 and 1.3) and dihydropyridine-insensitive N-type (
2.1), P/Q-type (
2.2), and R-type (
2.3) channels, which are distinguished by their single-channel conductance, pharmacology, and metabolic regulation. The second group is known as the low-voltage activated channels (
3.1, 3.2, and 3.3), or T-type channels, because they require less depolarization for their activation and their subsequent inactivation and a strong membrane hyperpolarization to bring them out of their steady inactivation (Catterall, 2000). Both L- and T-type
channels are expressed in pituitary cells (Stojilkovic et al., 2012).
A model for current through L-type channels includes only channel activation, although inactivation can occur on a slow time scale of seconds. The current is modeled as

where the Nernst potential used in the model is
. The activation variable
has first-order kinetics and the time constant
is voltage dependent and small, with a value of a few milliseconds over a large voltage range. In practice, one often assumes that activation is instantaneous, and replaces the current with

The advantage of using this rapid equilibrium approximation is that one differential equation, for , is removed. The steady-state activation Boltzmann function is shown in Figure 2.3a, with shape parameters given in the figure caption. Notice that this current is not activated at the resting potential, but begins to activate when the cell is depolarized to
mV or higher.

Figure 2.3 Gating properties of channels. (a) Steady-state activation function (
) for the L-type
current. Shape parameters are
and
. (b) Steady-state activation (
, black) and inactivation (
, red) functions for the T-type
current. Shape parameters are
,
,
, and
.
A model for the transient T-type current has both activation and inactivation factors. The activation is much faster than the inactivation, so we again use the rapid equilibrium approximation to remove the differential equation for the activation variable. The resulting expression is

and the inactivation variable has first-order kinetics. The time constant would most accurately be described as a function of voltage, but the essential dynamics are not affected by treating it as a constant (10 ms), as we do here. Removing nonessential complexities facilitates the understanding of the system, and is an approach we typically take.
The steady-state activation and inactivation functions for the model T-type current are shown in Figure 2.3b. The activation curve is left-shifted compared with that for L-type
channels. In fact, there is a window near the resting potential of the cell where this current is partially activated and not totally inactivated (between
and
mV in the model), so the current can depolarize the cell away from rest and toward the spike threshold.
2.3.3 Voltage-gated potassium channels
Voltage-gated (
) channels are widely expressed in excitable cells and play a critical role in membrane hyperpolarization during an action potential as well as in the propagation of action potentials. Electrophysiological studies have revealed that at least four functional classes of
channels exist: fast or rapidly activating delayed rectifier, slow delayed rectifier (including M channels), A-type
channels and the ether-a-go-go-gene-related (ERG) channels. The fast delayed rectifier is usually responsible for very short action potentials. Slow delayed rectifier channels are also involved in cell repolarization and, as indicated by their names, the gating kinetics of these channels is slow and the channels are noninactivating. A-type channels show rapid activation in the subthreshold range of membrane potential, fast inactivation and fast recovery from inactivation, and these channels contribute to the regulation of firing frequency in excitable cells (Hille, 2001). All four functional classes of
channels are expressed in endocrine pituitary cells (Stojilkovic et al., 2010).
A model for the current through rapidly activating delayed rectifier channels (M channels are not discussed further) accounts for voltage-dependent activation and no inactivation. The rapid equilibrium approximation is not used since the activation process is slow compared to
channel activation and this is crucial to the role of the current in action potential generation. The model current is

where the Nernst potential is very negative ( in the model). The activation variable has first-order kinetics, and we use
. The steady-state activation function is shown in Figure 2.4a. Note that it is right shifted relative to the
channel activation functions. This and the slower activation rate slow down the negative feedback provided by this current; delayed negative feedback is an important element in the generation of an action potential.

Figure 2.4 Gating properties of channels. (a) Steady-state activation function (
) for the delayed rectifier
current. Shape parameters are
and
. (b) Steady-state activation (
, black) and inactivation (
, red) functions for the A-type
current. Shape parameters are
,
,
, and
. (c) Steady-state activation function (
) for the inward rectifying
current. Shape parameters are
and
.
Like the T-type current, the A-type
current has fast activation, slower inactivation, and a window for which the current is partially activated and not entirely inactivated at steady state. However, there are some important differences between those two currents. First, the A-type current is hyperpolarizing, while the T-type current is depolarizing. Second, the steady-state activation window is right shifted for A-type versus T-type, so that the current plays little role in setting the rest potential. Instead, it is important in modulating action potential frequency. The model for the current is

where rapid equilibrium is used for the activation, and the inactivation variable has first-order kinetics with . The activation and inactivation functions are shown in Figure 2.4b.
2.3.4 Inwardly rectifying potassium channels
Inwardly rectifying potassium () channels produce an inward current under hyperpolarization, leading to
influx, with little
efflux under depolarization. Although the amplitude of the outward currents flowing through these channels is limited, they profoundly influence the resting membrane potential. There are 15 members of this family of channels that can be classified into three groups, based on their regulation patterns. Some of these channels are constitutively active at rest, leading to
leaking from cells, whereas the activity of others is influenced by certain modulators, such as G proteins and nucleotides. The long cytoplasmic pore of these channels plays a critical role for inward rectification, and it provides the structural basis for the modulation of gating by G proteins and phosphatidylinositol 4,5 bisphosphate (Stanfield et al., 2002). The mRNA transcripts for numerous
channels were identified in anterior pituitary cells. Functional studies have also indicated the presence of the constitutively active
-like channels and G protein-regulated
channels (Stojilkovic et al., 2010).
A model for the current is

where rapid equilibrium is assumed for the activation. The activation function, shown in Figure 2.4c, drops rapidly from 1 (fully activated) to 0 (fully deactivated) near the rest potential.
2.4 Nonselective cation channels
The majority of transient receptor potential (TRP) channels are relatively nonselective cation-conducting channels. Six protein families comprise the mammalian TRP superfamily: the ‘canonical’ receptors (TRPCs), the vanilloid receptors, the melastatin receptors, the polysistins, the mucolpins, and the ankyril transmembrane protein 1 (Clapham et al., 2005). The mRNA transcripts for TRPC1, TRPC3, TRPC4, TRPC5, and TRPC7 have been identified in pituitary cells. The presence of these channels in endocrine pituitary cells was indicated pharmacologically, and they may contribute to the excitability of these cells (Tomic et al., 2011).
We model the current through nonselective cation channels as a constant-conductance current with a reversal potential between and
. We use
.
2.5 Ligand-gated ion channels
Many types of ion channels respond to chemical signals (ligands) rather than (or in addition to) changes in the membrane potential and they are called ligand-gated ion channels. The largest group of these channels is activated by neurotransmitters (Collingridge et al., 2009). Endocrine pituitary cells express these channels, including GABA-gated channels and ATP-gated P2X channels, and their role in the excitability of pituitary cells is discussed elsewhere (Stojilkovic et al., 2010). Intracellular ligand-gated channels have an intracellular ligand-binding domain and interact with intracellular messengers, such as and cyclic nucleotides. Pituitary cells express
-activated
channels (Van Goor et al., 2001aa,2001bb) and hyperpolarization-activated cyclic nucleotide-gated channels (Kretschmannova et al., 2012), both located at the plasma membrane. The main function of these channels is to convert
and cyclic nucleotide signaling into electrical information. Other intracellular ligand-gated channels are located in intracellular membranes, such as the endoplasmic reticulum (ER). Pituitary cells express inositol trisphosphate (
) receptor channels, which are selectively permeable to
. In excitable cells, including pituitary cells,
release from the ER has a profound effect on excitability (see Chapter 3).
2.5.1 Calcium-controlled potassium channels
Calcium-controlled potassium () channels are composed of two distinct families. One family includes three small-conductance (SK) channels (
2.1, 2.2 and 2.3) and the other includes one intermediate-conductance (IK) channel (
3.1). They are voltage-insensitive, and the rise in the intracellular
concentration is critical for their activation. The other family consists of high-conductance
(BK) channels. They are activated by voltage, and their open probability is modulated by
. The BK channels, but not SK channels, form macromolecular complexes with
channels. This provides an effective mechanism for the control of the activity of these channels by
influx through
channels (Wei et al., 2005). Both SK and BK channels are present in endocrine pituitary cells (Van Goor et al., 2001bb).
A model for the SK- or IK-type current has a conductance that depends on the free concentration in the cytosol, rather than the voltage

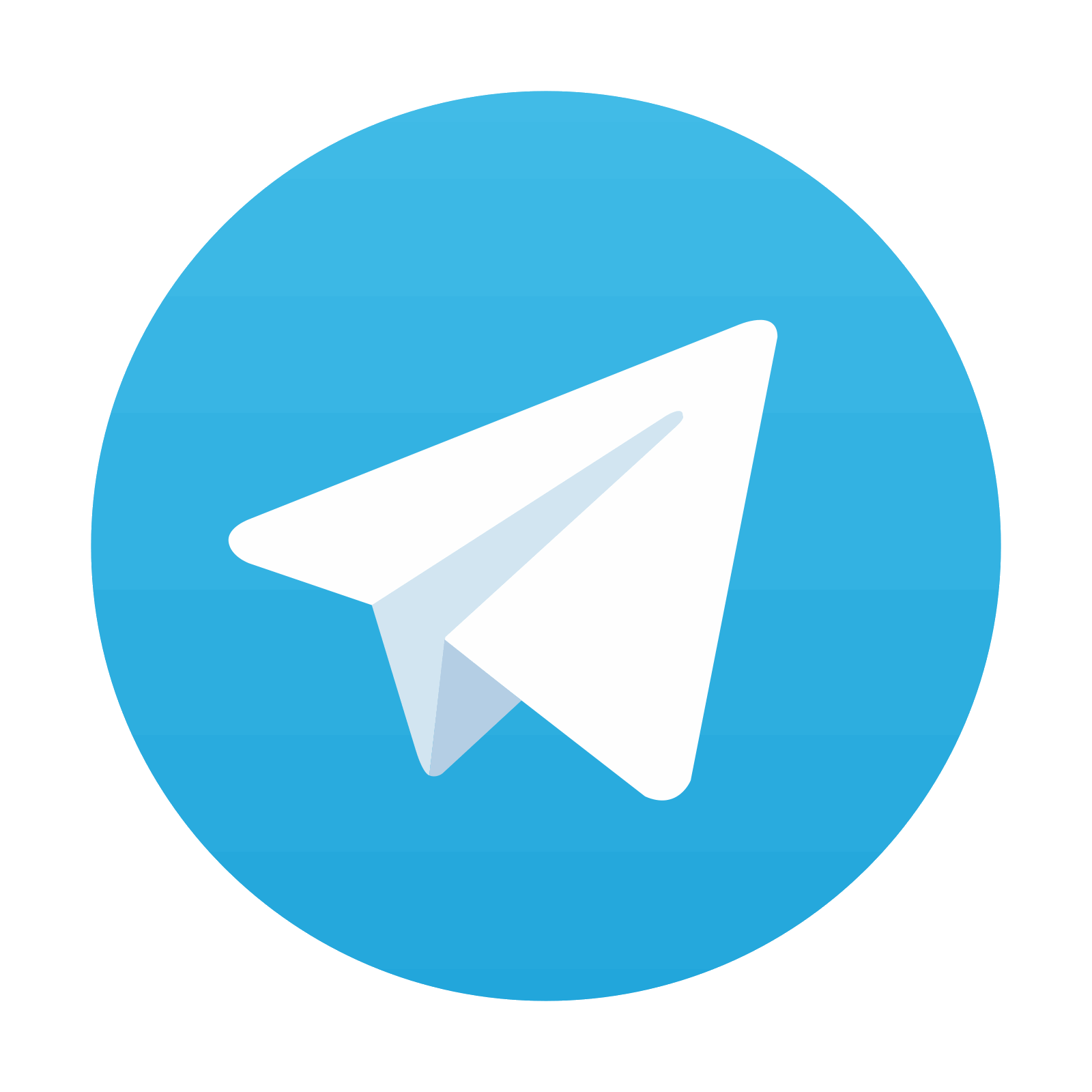
Stay updated, free articles. Join our Telegram channel
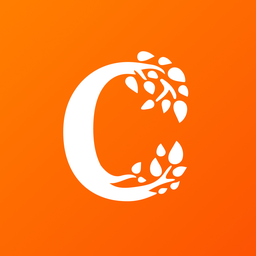
Full access? Get Clinical Tree
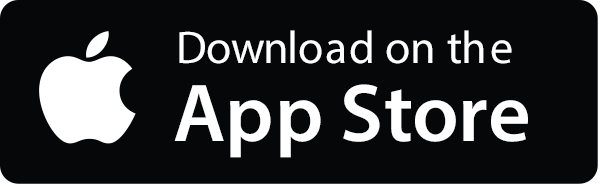
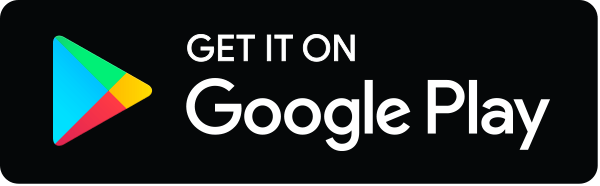