Fig. 22.1
Proposal of a hodotopical model of functional connectivity in the human brain, with incorporation of anatomical constraints. This networking model was elaborated on the basis of structural-functional correlations provided by intraoperative direct cortico-subcortical electrostimulation mapping
Posterior thalamo-cortical somatosensory pathways and their somatotopy have also been investigated by DEM, which generate dysesthesias or tingling in awake patients [39, 64]. Interestingly, axonal stimulation of the white matter behind the central sulcus may also induce disturbances in movement control [65], possibly due to transient inhibition of U fibers within the rolandic region [69]. Moreover, in patients who experienced interference with movement during subcortical DEM, fibers that induced inhibition or acceleration were located immediately posterior to thalamo-cortical somatosensory pathways. Therefore, a thalamo-parietal connection distinct from somatosensory pathways is likely [69]. On the basis of these original DEM findings, the existence of a wide fronto-thalamo-parietal sensory-motor network has been suggested [65, 69].
22.7.2 Visual Tract
The optic radiations arise from the lateral geniculate body in three bundles. The anterior bundle curves anterolaterally above the temporal horn (Meyer’s loop) usually reaching beyond the anterior limit of the temporal horn and then loops backward along the inferolateral wall of the atrium. The middle bundle courses laterally around and turns posteriorly along the lateral wall of the atrium and the occipital horn. The posterior bundle courses directly backward, also along the lateral wall of the atrium and occipital horn [70]. Recently, visual pathways have also been mapped in awake patients. A protocol was recently proposed in which two images, located in opposite quadrants on the same computer screen, are shown to the patient. It is possible to generate a transient visual field deficit subjectively described by the patient, which is confirmed objectively with this test (only one of the two objects can be seen and, thus, described) during axonal DEM of the optic radiations [40].
Interestingly, DEM can generate either “negative effect” such as blurred vision or impression of shadow, or “positive effect” such as phosphenes in the contralateral visual field. In addition, complex responses such as visual hallucinations (e.g., zoopsia or distortion of pictures as metamorphopsia) have been described by patients during stimulation [71]. These findings might be explained by the fact that DEM of bundles joining the calcarine fissure evokes visual suppression (homonymous hemianopia), whereas DEM of fibres joining the association visual cortex for higher-order visual processing (outside the primary visual system) evokes visual illusion.
22.7.3 Connectivity Mediating Language
On the basis of DEM findings, a dual model for visual language processing has recently been proposed, with a ventral stream involved in mapping visual information to meaning (the “what” pathway) and a dorsal stream dedicated to mapping visual information to articulation through visuo-phonological conversion [43]. These findings complete the seminal model by Hickok and Poeppel [61], which is a pure cognitive model that do not take into account anatomical constraints, especially with regard to the white matter tracts.
22.7.3.1 Functional Anatomy of the Dorsal Superior Longitudinal Fascicle (SLF)/Arcuate Fascicle (AF) Complex
Fiber dissection in cadavers and DTI tractography studies in humans have investigated the structural anatomy of the SLF/AF complex [72, 73]. The different components of the perisylvian SLF were isolated and the tracts were followed until their cortical terminations. Three segments of the perisylvian SLF were detected: (1) anterior segment of the lateral SLF, that connects the supramarginal gyrus and superior temporal gyrus (in the region just posterior to the Heschl’s gyrus) with the ventral portion of the precentral gyrus (ventral premotor cortex), (2) posterior segment of the lateral SLF, that connects the posterior portion of the middle temporal gyrus with the angular gyrus, and (3) long segment of the AF, deeply located, stemming from the caudal part of the temporal lobe, mainly the inferior and middle temporal gyri, that arches around the insula and advances forward to end within the frontal lobe, essentially within the precentral gyrus and posterior portion of the inferior and middle frontal gyri. Based on these original data challenging the traditional view, it was suggested that the fibers from the posterior part of the superior temporal gyrus are part of the anterior portion of the perisylvian SLF and not of the AF [72].
In awake patients performing a picture-naming task, cortically, phonemic paraphasias have been generated by DEM of the inferior parietal lobule and inferior frontal gyrus in the dominant hemisphere [74]. Axonally speaking, these phonemic paraphasias were evoked when stimulating the AF [74, 75], possibly associated with repetition disorders [76]. This is in agreement with the theory by Geschwind [77], who postulated that damages of this bundle would produce conduction aphasia, including phonemic paraphasias and repetition disturbances, and this supports the role of the sub-part of the dorsal stream mediated by the AF in phonological processing. Interestingly, the posterior cortical origin of the AF within the posterior part of the inferior temporal gyrus corresponds to the visual object form area [72]. Indeed, this region represents a functional hub, involved both in semantic and phonological processing dedicated to visual material [78, 79]. Therefore, phonological processing sustained by the AF is performed in parallel to the semantic processes implemented by the ventral route (see below). In addition to this direct dorsal route, the indirect dorsal stream composed of the lateral SLF is involved in articulation and phonological working memory, as demonstrated by DEM. Cortical areas eliciting articulatory disorders are located in the ventral premotor cortex, supramarginal gyrus and posterior part of the superior temporal gyrus [80, 81]. Axonally, stimulation of the white matter under the fronto-parietal operculum and supramarginal gyrus, laterally and ventrally to the AF, elicited anarthria as well [74, 81]. This bundle corresponds to the part III of the SLF according to Makris et al. [82]. Indeed, this lateral operculo-opercular component of the SLF constitutes the articulatory (auditory-motor) loop, by connecting the supramarginal gyrus/posterior portion of the superior temporal gyrus (which receives feedback information from somatosensory and auditory areas) with the frontal operculum (which receives afferences bringing the phonological/phonetic information to be translated into articulatory motor programs and efferences toward the primary motor area) [72, 76, 81].
Using the same paradigm, DEM also supported that syntactic processing was mediated by delocalized cortical regions (including left inferior frontal gyrus and posterior middle temporal gyrus) connected by a sub-part of the left SLF. Interestingly, this sub-circuit is interacting but independent of the sub-network involved in naming, as demonstrated by a double dissociation between syntactic (especially grammatical gender) and naming processing during DEM. These findings support a parallel rather than serial theory, calling into question the principle of “lemma” [83].
22.7.3.2 Functional Anatomy of the Ventral Route
The ventral stream connects the occipital, parietal and posterior temporal areas with the frontal lobe. This ventral route is referred by some authors as “extreme capsule fiber system” with reference to connectivity studies in the primate [84]. It seems nonetheless more adapted to talk about fascicles rather than “extreme capsule”, because the latter only considers a discrete anatomical structure while the former considers actual neural pathways with their cortical termination, in a connectomal view of brain processing. Indeed, if one takes account of the sole subcortical region without any considerations regarding the cortical epicenters connected by these white matter fibers, this does not allow the understanding of the whole eloquent network.
Regarding structural anatomy, the ventral route is composed of direct and indirect pathways. The direct pathway is represented by the IFOF, that has never been described in animals, explaining the controversy about its role [46]. In humans, the IFOF is a ventral long-distance association bundle that connects the occipital lobe, parietal lobe and the postero-temporal cortex with the frontal lobe. Recent anatomic dissections combined with DTI have investigated the main course of the IFOF [85, 86]. From the posterior cortex, it runs within the sagittal stratum in the superior and lateral part of the atrium; it reaches the roof of the sphenoidal horn in the temporal lobe; it joins the ventral part of the external/extreme capsule and it runs under the insula at the posterior two-thirds of the temporal stem; then it joins the frontal lobe [85]. Two layers of the IFOF have been described [86]. The superficial and dorsal layer connects the posterior portion of the superior and middle occipital gyri, the superior parietal lobule and the posterior part of the superior temporal gyrus to the inferior frontal gyrus (pars triangularis and opercularis). The deep and ventral subcomponent connects the posterior portion of the inferior occipital gyrus, the posterior temporal-basal area including the Fusa (fusiform area at the occipito-temporal junction) and the posterior part of the middle temporal gyrus to the frontal lobe – orbito-frontal cortex, middle frontal gyrus and dorsolateral prefrontal cortex [85, 86].
In parallel, the ventral stream is underpinned by an indirect pathway, constituted by the anterior part of the inferior longitudinal fascicle (ILF) (running below the IFOF), that links the posterior occipitotemporal region (Fusa) and the temporal pole (TP), then relayed by the uncinate fasciculus (UF), that connects the TP to the basifrontal areas by running within the anterior third of the temporal stem (in front of the IFOF) [85]. Of note, the posterior part of the ILF links the occipital lobe to the posterior occipitotemporal junction (visual object form area) [70, 79]. This means that this indirect route connects the occipital/Fusa to the orbito-frontal cortex – thus partially overlapped with the IFOF. Finally, while previously described in monkey, another pathway has recently been observed in humans, the middle longitudinal fascicle (MdLF), that connects the angular gyrus with the superior temporal gyrus up to the TP and courses under the superior temporal sulcus, lateral and superior to the IFOF [89].
In the awake patient, during picture naming, DEM of the IFOF, at least in the dominant hemisphere, elicited semantic paraphasias either associative (e.g. /key/ for /padlock/) or coordinate (e.g. /tiger/ for /lion/) in more than 85% of cases [90]. It did not matter what portion of the IFOF was stimulated (parieto-occipital junction, temporal, subinsular or frontal part) [43, 56]. These language disorders were mainly induced by stimulating the superficial layer of the IFOF. Interestingly, semantic paraphasias were never observed during stimulation of the dorsal route (SLF) [74]. Moreover, IFOF stimulation may also generate verbal perseveration [91], raising the question of its role in semantic control.
The functional role of the indirect ventral pathway is still debated. On one hand, this indirect route connects areas involved in verbal semantic processing such as Fusa and lateral frontal cortex [92]. Moreover, the major cortical relay between the ILF and UF is the TP, which is a hub, i.e. a functional epicenter enabling a plurimodal integration of the multiple data coming from the unimodal systems (subserved by ILF, UF and MdLF), explaining its role in semantics and its implication in semantic dementia when bilaterally damaged [93]. On the other hand, except for the posterior part of the ILF which is involved in visual recognition and reading [78, 79, 87, 88] and for which injury generates alexia [78, 79], the indirect pathway can be functionally compensated when unilaterally damaged [94–96]. This was also confirmed by language recovery following anterior temporal lobectomy in tumor and in epilepsy surgery [94, 97]. Even if very mild and selective deficit may persist, as concerning proper name retrieval after resection of the UF [98], or a more difficult lexical access after resection of the anterior part of the ILF combined with resection of the posterior part of the inferior temporal cortex [99], this is a good illustration of the concept of “subcortical plasticity”, in which a sub-network (IFOF, direct pathway) is able to bypass another sub-network (indirect pathway) and to functionally compensate it [14, 96]. Similarly, DEM of MdLF and resection of its anterior part failed to induce any functional disorders [100], demonstrating that this fascicle converging to the TP can also be compensated.
In summary, DEM has enabled a re-examination of the classical Broca–Wernicke localizationist model of language. The new findings from axonal stimulation provide a distributed framework for future studies of language networks and for management of patients with aphasia. This new model is based on multiple direct and indirect corticosubcortical interacting subnetworks involved in syntactic, semantic, phonological and articulatory processes. It offers several advantages in comparison with previous models, especially by explaining double dissociations during damage of the ventral versus dorsal stream (semantic and phonemic disorders, respectively). Also, it takes into account the cortical and subcortical anatomical constraints [43].
22.7.4 Connectivity Sustaining Visuospatial and Vestibular Processing
In awake patients, axonal DEM of the ILF has generated contralateral visual hemiagnosia, supporting the existence of an occipitotemporal pathway connecting occipital visual input to higher-level processing in temporal lobe structures, in particular the fusiform gyrus [101]. These stimulation findings support a crucial role for the ILF in visual recognition, with specialization of this bundle for visuospatial processing in the right hemisphere and language processing in the left hemisphere.
Stimulation of a specific part of the SLF (called the SLF II) in the right hemisphere can produce spatial cognition problems. Indeed, DEM of this structure elicited rightward deviation in a line bisection test [42]. These data suggest that parietal–frontal communication is necessary for symmetrical processing of the visual scene. In other words, spatial awareness depends not only on the cortical areas of the temporal–parietal junction, but also on a larger parietal–frontal network communicating via the right SLF.
Finally, stimulation of another subcircuit in the right SLF can cause a central vestibular syndrome with vertigo, by disrupting the vestibular inputs assembled in the temporoparietal areas and the prefrontal cortex [41]. This finding demonstrates the role of the SLF in the network coordinating body posture and spatially oriented.
22.7.5 Connectivity Subserving Mentalizing
Regarding the network sustaining mentalizing, namely, the theory of mind, crucial for emotion and social cognition, intraoperative DEM (that can interfer with the neural activity of mirror-related frontal areas by impairing mentalistic inferences) [47], combined with pre- and post-operative behavioral examinations showed that this function is made possible by parallel functioning of two subsystems. The first, low-level, of mentalizing accuracy of identification (mirror system, i.e. the ability to appreciate other people’s emotions) is subserved by the AF/SLF complex; the second, high-level of inferential mentalizing, corresponding to the attribution of mental states, is mediated by the cingulum [102, 103]. These findings, which constitute the first experimental data on the structural connectivity of the mentalizing network, suggest the existence of a dual-stream connectomal model, and could lead to a better understanding of disorders that affect social cognition, as autism [102].
22.7.6 Fibers Underlying Cognitive Functions
DEM also evidenced the existence of an executive system (including prefrontal cortex, anterior cingulate and caudate nucleus) involved in the cognitive control of more dedicated subcircuits, as for example the subnetwork involved in language switching—itself constituted by a wide cortico-subcortical network comprising postero-temporal areas, supramarginal and angular gyri, inferior frontal gyrus and a sub-part of the SLF [104]. Moreover, the frontal aslant tract, that connects the pre-supplementary motor area and anterior cingulate with the inferior frontal gyrus, seems to play a role in language control, especially with regard to planning of speech articulation [66]. Therefore, DEM of this tract may evoke stuttering [105]. In the same vein, a cortico-subcortical loop involving the deep grey nuclei, especially the caudate nucleus, was also demonstrated as participating in the control of language (selection/inhibition), since DEM of the head of the caudate nucleus in the left hemisphere generated perseverations with a high level of reliability [106]. Anatomically, this cortico-striatal loop seems to be supported by the fronto-striatal tract [66].
DEM of the IFOF also induced non-verbal comprehension disturbances during non-verbal semantic association test—e.g. Pyramid and Palm Trees Test. The patients were not able anymore to make a semantic choice during DEM, with some of them still able to join a short verbal description of their feelings, like “I don’t know at all”, “what do I have to do?”, “I don’t understand anything” [46]. These comprehension disorders were mainly generated by stimulating the deep layer of the IFOF, and elicited a double dissociation: semantic paraphasia with normal non-verbal semantic choice during DEM of superficial IFOF and vice versa during DEM of deep IFOF. Thus, it was suggested that the existence of a superficial component involved in verbal semantics (see above) and a deep component involved in amodal semantic processing [46].
These data are in agreement with the cortical terminations of the IFOF (prefrontal, temporal-basal and parietal areas), that correspond with the cortical network involved in semantic control [86]. Consequently, from the new insights gained from axonal DEM, an original anatomo-functional model of semantic processing has been recently proposed, in which the crucial pathway is represented by the IFOF. In this model, visual information is processed at the level of the occipital and temporal-basal associative cortices, and auditory information is processed at the level of the temporal and parietal associative cortices. They are transmitted directly on an amodal shape to the prefrontal areas, which exert a top down control over this amodal information in order to achieve a successful semantic processing in a given context. DEM of this fascicle generates a disruption of these rapid direct connections. The transient semantic disorganization observed when stimulating the IFOF would therefore be caused by a dis-synchronization within this large-scale network, interrupting simultaneously the bottom up transmission and the top down control mechanisms [46]. Thus, IFOF might play a crucial role not only in verbal and non-verbal semantic processing, but also in the awareness of amodal semantic knowledge, namely noetic consciousness. From a phylogenic perspective, because recent studies in the primate failed to identify this tract, one could suggest that the IFOF is the proper human fascicle. This multi-function fascicle allows human to produce and understand language, to manipulate concepts, to apprehend and understand the world (i.e. metalinguistics, conceptualization and awareness of knowledge) and it contributes to make the human what he is, with his infinite wealth of mind [46].
In the same spirit, axonal DEM has shown that disruption of the subcortical connectivity of the left posterior cingulate cortex reliably induced a breakdown in conscious experience [48, 49]. This acute phenomenon was mainly characterized by a transient behavioral unresponsiveness with loss of external connectedness (the patients described themselves as in a dream, outside the operating room). This finding suggests that functional integrity of posterior cingulate connectivity is necessary to maintain consciousness of the external environment. In other words, axonal DEM can open the door to the investigation of the connectomal anatomy underlying distinct levels of awareness, including the pathways involved in monitoring of the human level of consciousness related to semantic memory (noetic consciousness), as well as the pathways that sustain consciousness of the external world. Axonal DEM can also be used to explore the dynamic interactions between these subcircuits, and possible functional regulation of the intrinsic activity of these subcircuits by other subnetworks, such as the cingulothalamic system [48].
To sum up, these original insights provided by DEM strongly support the fact that cerebral functions are underpinned by extensive circuits comprising both the cortical epicenters and connections between these “hubs”, created by associating bundles of white matter [107]. In this hodopical model challenging the traditional localizationist view, neurological function comes from the synchronization between different epicenters, working in phase during a given task, and explaining why the same hub may take part in several functions depending on the other cortical areas with which it is temporarily connected at any one time. One step forward, it is crucial to consider that complex brain processes are possible only because of dynamic interactions exist between these parallel delocalized sub-networks, with different levels of sub-circuits recruitment according to the task required. Therefore, brain processing should not be conceived as the sum of several subfunctions. Rather, cerebral function results from the integration and potentiation of parallel (while partially overlapped) subnetworks, in a connectomal view of CNS functioning [33].
22.8 Cerebral Reshaping: Its Implications for (Surgical) Treatment of DLGG
Maximal surgical resection is the first option in DLGG (see next chapter). Consequently, it is important to be reliable in the preoperative estimation of the extent of resection. Interestingly, such prediction will directly depend on the involvement (or not) of subcortical pathway which cannot be functionally compensated (see probabilistic atlases of functional plasticity described above)—and this will lead to the selection of surgery as a first treatment or in contrast to give neoajuvant chemotherapy if the gliomas is too diffuse within the “minimal common brain” (see chapter by Duffau and Taillandier) [24]. Results of the neuropsychological assessment performed before any treatment will also participate in the investigation of the individual plastic potential. In other words, if the patient already experienced significant cognitive disorders and a fortiori neurological deficit, this means that limits of brain plasticity have been reached—preventing functional compensation. Such parameters should be incorporated in the surgical strategy with the goal (1) to extent the indications of resection in eloquent structures so far considered as “inoperable” (2) to maximize the extent of glioma removal, by performing the resection according to individual functional boundaries with no margin (3) while minimizing the risk of postoperative permanent neurological worsening or even by improving the quality of life [1, 30].
Thanks to the phenomena of preoperative and intraoperative plasticity, several surgical series showed that it was possible to undertake wide surgical resection of DLGG invading regions of the brain conventionally deemed to be inoperable. Extensive DLGG removals have therefore been carried out without causing permanent neurological deficits in the following “eloquent” brain structures (Fig. 22.2).
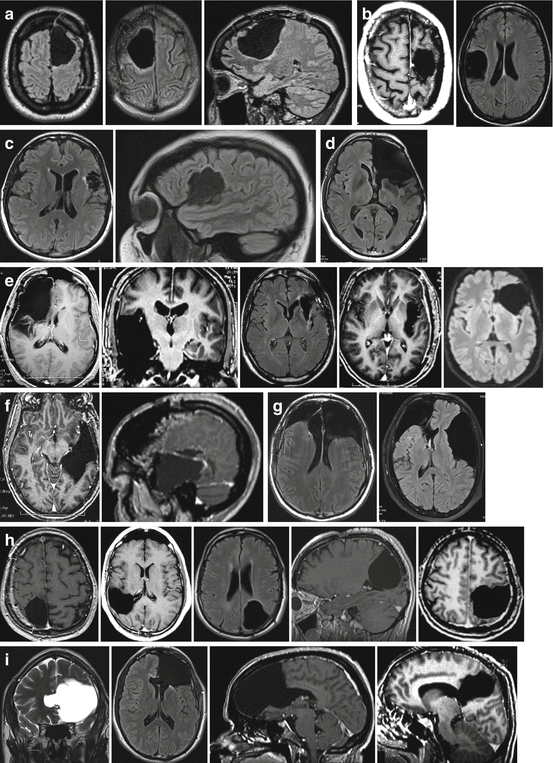
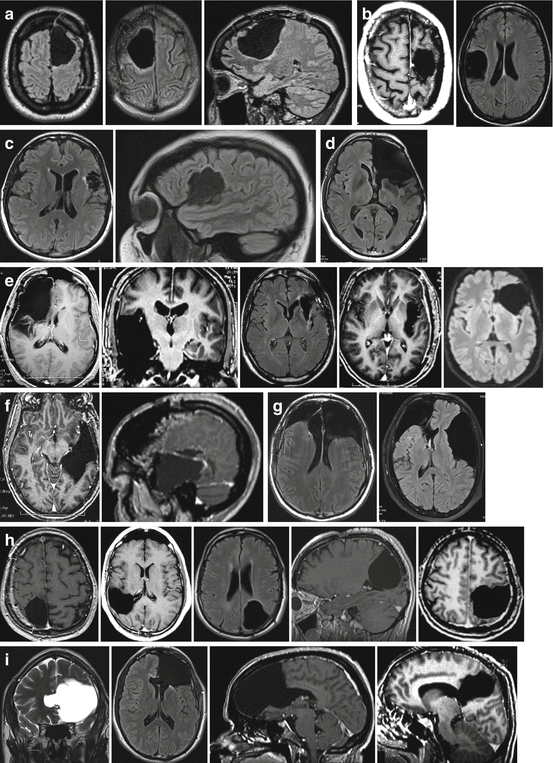
Fig. 22.2
Examples of extensive glioma resections performed within the so-called “eloquent” areas using intraoperative electrical mapping, with preservation of the quality of life thanks to brain plasticity: (a) left and right supplementary motor areas; (b) left primary motor area of the hand (“knob of the hand”) and right primary sensory-motor area of the face; (c) “Broca’s area” and the rolandic operculum in the left dominant hemisphere; (d) entire left frontal lobe including “Broca’s area”; (e) right paralimbic system, left insula and left operculo-insular complex; (f) left dominant temporal lobe, including “Wernicke’s area” (posterior to the Labbé vein); (g) bilateral lesions; (h) parietal lobe in right (superior and inferior parietal lobule) and left hemispheres, including the primary somatosensory area; (i) corpus callosum, anterior or posterior part (splenium)
22.8.1 Resection of DLGG Within Broca’s Area
Surgical resection of DLGG within the pars opercularis and/or triangularis of the left inferior frontal gyrus can be performed without generating permanent language deficits [108–111]. Language compensation may be underlain by the recruitment of adjacent regions, primarily the ventral premotor cortex (vPMC), the pars orbitaris of the inferior frontal gyrus, the dorsolateral prefrontal cortex and the insula [108]. Indeed, it has recently been demonstrated that the left vPMC (located behind the pars opercularis) cannot be (totally) removed because it was in fact the final common pathway for speech – while Broca’s area can be compensated after resection [81, 112]. Indeed, extensive neuropsychological examination following resection of Broca’s area confirmed a complete functional recovery [109]. A transopercular surgical approach through Broca’s area, even though not invaded by the tumor, has even been reported in insular DLGG in order to avoid splitting the Sylvian fissure and therefore reducing the risk of vascular injuries: no persistent language deficits have been observed [113, 115]. Interestingly, a recent probabilistic map for crucial cortical epicenters of human brain functions, based on over 700 DEM data obtained in 165 consecutive patients who underwent awake mapping for DLGG resection, challenged the classical theories of brain organization by demonstrating that Broca’s area is not the speech output area [115, 116].
22.8.2 Resection of DLGG Within Wernicke’s Area
The language compensation following resection of DLGG involving the posterior part of the left “dominant” superior temporal gyrus (and its junction with the inferior parietal lobule) could be explained by the fact that this complex function is organized in multiple parallel networks. As a result, in addition to the recruitment of areas immediately adjacent to the surgical cavity (e.f. the supramarginal gyrus), the long term reshaping could also involve progressive recruitment of remote regions within the left dominant hemisphere—such as the pars triangularis of inferior frontal gyrus or other left frontolateral regions—as well as controlateral sites in the right hemisphere because of transcallosal disinhibition [117]. Indeed, a recent study combining functional MRI and DEM evidenced the existence of a wide bilateral cortico-subcortical network able to compensate surgical resection of left Wernicke’s area invaded by glioma [118].
22.8.3 Resection of Insular DLGG
Despite a possible hemiparesis after right insular DLGG removal, likely because this region is a non-primary motor area, and possible transient speech disturbances following left dominant insular DLGG resection, all patients recovered in a personal experience—except in rare cases (2%) of deep stroke due to a damage of the lenticulo-striate arteries [114, 119–121]. QoL was even improved in about a third of patients who had epilepsy which was refractory to preoperative medical treatment, and in whom removal of the insular tissue (and even when necessary additional removal of the temporomesial structures), controlled the seizures. Moreover, it has been possible to remove the claustrum with no cognitive disorders (despite its role suggested in consciousness) in right non-dominant fronto-temporo-insular DLGG involving the deep grey nuclei [122]. The right striatum was also resected when invaded by DLGG without causing motor deficit or movement disorders, even after a long-term follow-up over 10 years: this compensation can likely be explained by a recruitment of parallel subcortical circuits such as pallido-luyso-pallidal, strio-nigro-striate, cortico-strio-nigro-thalamo-cortical and cortico-luysal networks [123].
22.8.4 Resection of DLGG Involving the Primary Sensory-Motor Area of the Face
Despite transient cerebral facial paralysis, the bilateral hemispheric cortical representation of this function explains why all patients have recovered [124]. However, if the insula is also invaded, a transitory Foix-Chavany-Marie syndrome may be produced with transient bilateral transient orofacial pharyngeal laryngeal paralysis [125].
22.8.5 Resection of DLGG Involving the Primary Motor Area of the Upper Limb
The “rigid” somatotopic organization of the homunculus needs to be replaced by a more dynamic vision of the functioning of sensorimotor areas, based on the fact that there are redundancies, both within the primary motor cortex and between the pre-and retrocentral regions (see above). Stimulation of the precentral gyrus can regularly produce somatosensory responses, whereas stimulation of the retrocentral gyrus may lead to involuntary muscle contractions or even to stop movement—indicating the existence of a complex central network and not a succession of discrete independent sites [53]. Unmasking of the latent subnetworks can therefore be demonstrated in real time in the operating theater, allowing sensorimotor maps to be reorganized within an hour and permitting more complete resection without causing deficit: these are short term plasticity mechanisms [54, 55]. Moreover, long-term redistribution effects (such as years later after the first operation) may also occur, optimizing tumor resection during a reoperation compared to the initial surgery, including surgery in the”knob of the hand” [117, 125, 126] (see below).
22.8.6 Resection of DLGG Within the Primary Somatosensory Area
Due to a dynamic organization of the whole central region referred to above, results using pre- and post-operative functional neuroimaging have suggested the possible recruitment of “redundant” eloquent sites around the cavity, within the postcentral gyrus, after removal of a DLGG involving the primary somatosentory cortex. This is in accordance with the intraoperative DEM data, showing unmasking of redundant somatosensory sites during resection, likely explained by the decrease of the cortico-cortical inhibition. In addition, recruitment of secondary somatosensory areas and/or posterior parietal cortex, primary motor area (due to strong anatomo-functional connections between the pre- and retro-central gyri), and controlateral primary somatosensory area may contribute to functional compensation to various degrees [117, 127].
22.8.7 Resection of DLGG Involving the Supplementary Motor Area
This resection generally causes a typical syndrome, with transient symptoms of akinesia (which may be complete) and mutism (particularly after surgery to the left supplementary motor area), which improves rapidly over around 10 days and then resolves after a few weeks (usually after intensive rehabilitation) [128, 129]. Pre- and postoperative task-based and resting-state funtional MRI has shown recruitment of the supplementary motor area and contralateral premotor cortex, as well as a modulation of the intra-hemispheric and inter-hemispheric connectivity, contributing to recovery [130, 131]. A more detailed cognitive examination however may reveal persistent subtle but objective long-term problems, particularly with complex movements and bimanual coordination [130]. For this reason, preservation of networks subserving movement control may be considered in some patients in order to preserve an excellent quality of life (in a pianist for example) [38, 65–67].
22.8.8 Resection of DLGG Involving a Subsection of White Matter Tracts
Interestingly, while neuroplasticity is constrained by subcortical connectivity (see above), some parts of white matter pathways can be resected with no severe permanent functional deficit. Indeed, this overall picture is more complex in some respects, as witnessed by the results of tract-based cluster analyses reported in a recent probabilistic atlas of functional plasticity [6]. In this study, the vast majority of individual tracts were generally divided into a subsection with a low and a subsection with a higher functional compensation index—suggesting that groups of fibres within the same tract differ in their neuroplastic potential. This confirms observations made in clinical practice. A telling example is the ILF, which connects the temporal pole to the occipital cortex and the occipitotemporal junction. Although the posterior part of the ILF is always functional for a set of cognitive processes (including reading aloud [79] and visual recognition [78]), its anterior part appears to abandon its functional role once infiltrated by a DLGG. These gradients of plasticity in the basal inferotemporal system can be analysed with regard to the connectivity patterns in the occipitotemporal area. In addition to projections from the ILF, this region receives widespread neural connections from the posterior segment of the SLF, the IFOF and the AF or even the vertical occipital fasciculus. Accordingly, one can speculate that the information broadcast by the ILF towards the temporal pole under normal circumstances could be redistributed via other connectivities if functional compensation is prompted by glioma growth [94, 96]. In the same vein, a part of the corpus callosum invaded by DLGG may also be removed with no morbidity [132].
However, as previously mentioned, in spite of these rare exceptions, the axonal connectivity should be preserved in the vast majority of cases, to allow the occurrence of neuroplasticity mechanisms within a wide bilateral cortico-subcortical circuit—also involving the deep grey nuclei and cerebellum, as recently evidenced by means of resting-state functional MRI before and after surgery for DLGG [133].
22.9 Postoperative Plasticity Evidenced by Serial Mapping: Towards a Multi-Stage Surgical Approach
Beyond preoperative and intraoperative reshaping of brain networks, postoperative plasticity also accounts for the resectability of areas for a long time thought as “unresectable”. Again, these areas should be considered as nodes within a wide network: after their removal, the whole functional network will self-reorganize by dynamical and biological plasticity, and the function will finally be preserved. Indeed, the good clinical status 3 months after surgery (as evidenced by extensive neuropsychological testing) as well as the return to a normal life (including the return to work) in DLGG patients, argue for efficient plasticity mechanisms for these areas [2, 13, 18]. Such mechanisms induced by surgical resection within eloquent areas were also studied, by performing postoperative functional neuroimaging once the patient has recovered his preoperative functional status. In particular, several patients were examined following the resection of gliomas involving the supplementary motor area, which elicited a transient postsurgical syndrome (see above). Functional MRI showed, in comparison to the preoperative imaging, the occurrence of activations of the supplementary motor area and premotor cortex contralateral to the lesion: the contrahemispheric homologous thus participated to the post-surgical functional compensation [130, 131].
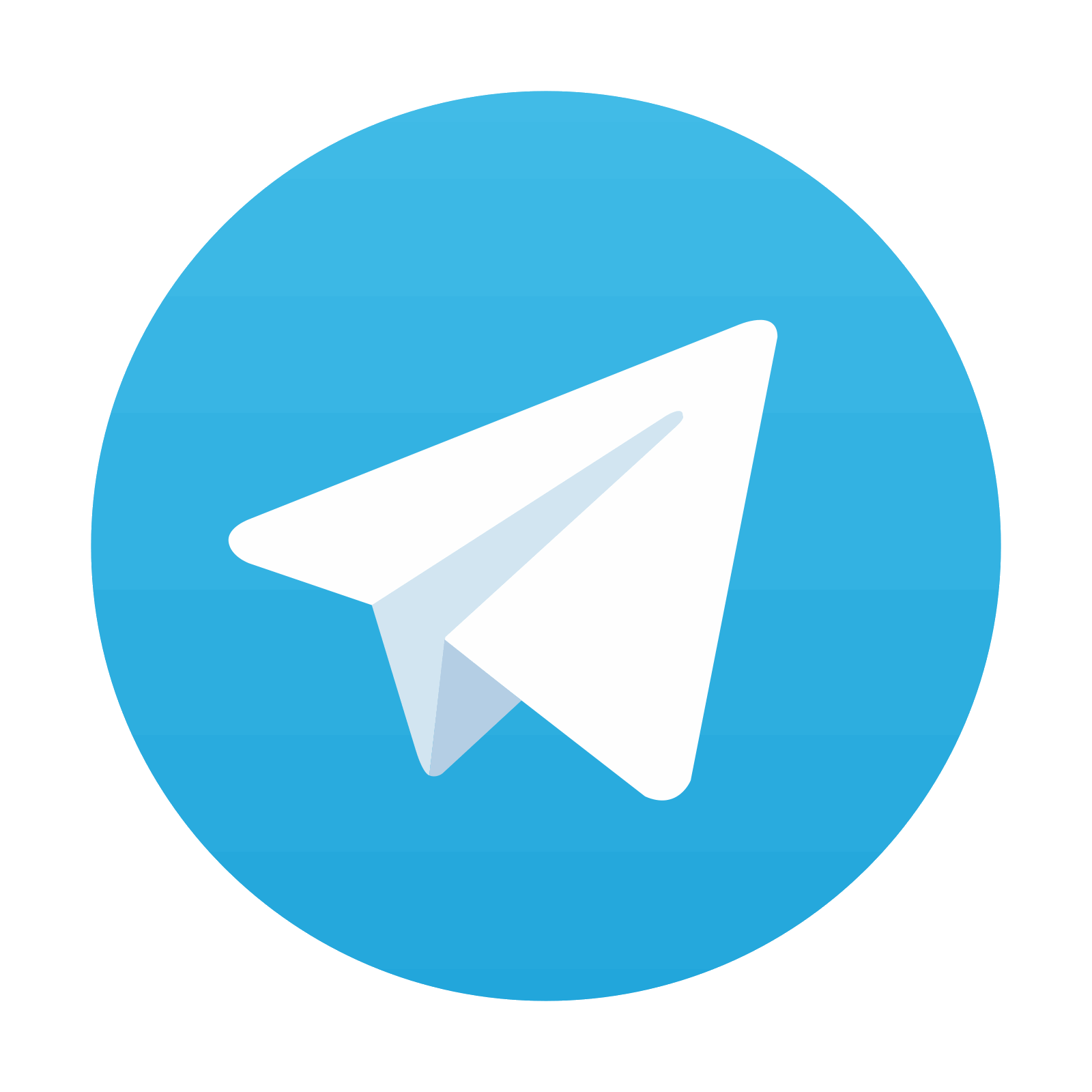
Stay updated, free articles. Join our Telegram channel
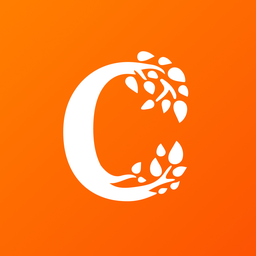
Full access? Get Clinical Tree
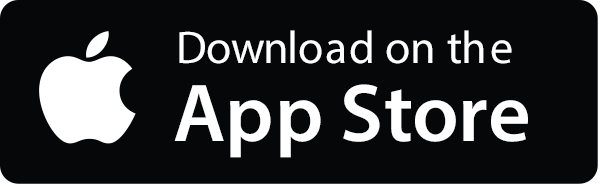
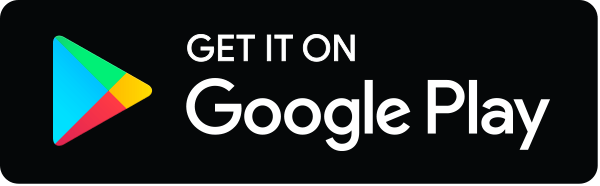