© Springer International Publishing AG 2018
Laurence Zitvogel and Guido Kroemer (eds.)Oncoimmunologyhttps://doi.org/10.1007/978-3-319-62431-0_2525. Innate Immune Receptors in the Regulation of Tumor Immunity
(1)
Department of Molecular Immunology, Institute of Industrial Science, The University of Tokyo, Komaba 4-6-1, Meguro-ku, Tokyo 153-8505, Japan
(2)
Max Planck-The University of Tokyo Center for Integrative Inflammology, Komaba 4-6-1, Meguro-ku, Tokyo 153-8505, Japan
Keywords
Innate immune responsePattern recognition receptorTumor developmentCytokinesInflammation25.1 Introduction
Cancer immunosurveillance, or the process by which the immune system searches and recognizes cancerous and cancer cells in the body, has a history going back to the early twentieth century when Paul Ehrlich predicted that the immune system represses the growth of carcinomas. The concept of cancer immunosurveillance has since been elaborated and contentiously debated by immunologists over many decades. After much aspiration and frustration, experimental evidence now unequivocally shows that such immune responses are indeed critical for the host defense against cancers [1–3]. Related to this are the interesting observations that the innate and adaptive immune systems contribute to the antitumor effects of conventional chemotherapy- and radiotherapy-based cancer treatments with such effects thought to be mediated at least in part through activation of innate immunity by the exposure of tumor-derived antigens during these treatments [4]. Thus, these classical cancer therapies are intimately tied to anticancer immune responses of the host.
The development of new and effective methods that harness the power of the immune system to treat cancers has become a very attractive and intense field of research. Recent years have seen remarkable progress in cancer immunotherapies including tumor-targeting monoclonal antibody, immune cell checkpoint therapy, tumor vaccine, and chimeric antigen receptor (CAR) T cell therapies [5–7]. While these therapies have brought about remarkable and in some cases durable response rates across many types of malignancies, the numbers of patients that benefit are still limited. As such, new therapies and combinations of existing ones that can potentiate the host’s antitumor immune responses with minimal or manageable adverse effects remain the focus in the field [8, 9].
The innate immune system employs many of the same mechanisms for the host defense against invading pathogens as it does in the eradication of cancer or cancerous cells [10, 11]. Perhaps the most well-studied cell type in these processes is the natural killer (NK) cell [12, 13]. In the context of anticancer innate responses, NK cells can directly kill virus-infected and cancerous cells. Indeed, animal models with impaired or deficient NK cells display increased incidences of various types of cancer [14]. Additional innate cell types including monocytic- and granulocytic-derived cells are known also to regulate, either positively or negatively, the antitumor adaptive immunity within and without the tumor microenvironment (TME) [15, 16]. In parallel to cellular immunity, the importance that the signal-transducing innate immune receptors play to the regulation of adaptive immune responses [17] has increased the attention on these receptors for their role in the regulation of oncogenesis [11]. Relative to the T cell receptor and B cell receptor, PRRs are a limited number of germline-encoded receptors that recognize pathogen-associated molecular patterns (PAMPs) or endogenous stress signals termed damage-associated molecular patterns (DAMPs) [11, 18]. As such, PRRs are not as discriminative as those for T cells and B cells [18–20].
Pattern recognition receptors (PRRs) have been subdivided into (1) membrane-associated PRRs, which include toll-like receptors (TLRs) and C-type lectin receptors (CLRs); (2) cytoplasmic PRRs, which include the RNA-sensing retinoic acid-inducible gene-I (RIG-I)-like receptor (RLR) family and DNA-sensing receptors; and (3) soluble PRRs, which include complement receptors, collectins, pentraxin proteins, and others [18–21]. The first two PRRs classes, but not the soluble PRRs, can transmit signals to the nucleus upon engagement with their cognate ligand(s) to alter gene expression profile of the cell. Most typically, this involves the induction of type I interferon (IFN) genes and inflammatory cytokine genes for mediating antimicrobial responses [18, 20, 22]. The innate signaling also directly drives dendritic cell (DC) maturation, antigen presentation, as well as cytotoxic CD8+ T cell functions [18–20].
There is accumulating evidence that antitumor immunity is regulated by the activation of PRRs [23]. Stimulation of PRRs by exogenously administered ligands induces robust antitumor immune responses in animal models [24–26], while excessive tumor growth has been observed in some PRR-deficient mice models [27, 28]. On the other hand, that the activation of PRRs evokes inflammatory responses raises the question of whether PRRs may actually hinder cancer immunity as the progression of some types of tumors is often associated with inflammation [29, 30]. The chronic activation of innate PRRs by endogenous ligands released from tumor cells may promote tumor progression through pro-inflammatory responses, which augments the proliferative, anti-apoptotic, and pro-fibrogenic signals within the TME [23, 29]. These seemingly contradictory reports indicate that innate immune activation by PRRs confers a dual role, i.e., an immune-enhancing role that potentiates antitumor immune responses and a tumor-promoting role through the induction of chronic inflammation.
In this review, we summarize our current understanding of innate PRRs which play a role in tumor immunosurveillance and regulation of oncogenesis. We also discuss the potential therapeutic implications of targeting tumors using modulators of the signal-transducing innate PRRs. For those interested in the broader biology of innate immune cells and PRRs, we recommend excellent reviews written by others [4, 11, 31, 32].
25.1.1 Role of TLRs in the Regulation of Tumor Development
The TLR family of receptors are by far the best understood classes of innate signaling PRRs which function as sentinels of pathogen infection [19, 20, 33]. TLRs can recognize microbial PAMPs and signal through recruitment of cognate adaptor proteins [34]. In general, TLRs commonly utilize for their signaling pathway the adaptor called myeloid differentiation primary response gene 88 (MyD88); however, TLR3 instead utilizes the TIR domain-containing adaptor-inducing IFN-β (TRIF, also called TICAM) signaling pathway, although there is evidence that it also utilizes the MyD88 pathway [34, 35]. TLR4 requires both MyD88 and TRIF pathway for full-blown activation of the downstream signaling cascades [34]. Upon binding to TLRs, the adaptor proteins engage additional downstream proteins that mediate the activation of transcription factors and protein kinases, such as nuclear factor-kappa B (NF-κB), IFN regulatory factors (IRFs), and mitogen-activated protein kinase (MAPK) to modify transcription of target genes such as those for type I IFNs and inflammatory cytokines [20, 34].
That TLRs are highly expressed in antigen-presenting cells and the activation of some TLRs induces antitumor mediators such as type I IFNs led to efforts to harness TLR agonists for cancer therapies. In fact, TLRs are prominent therapeutic targets for the activation of anticancer immune responses, originally highlighted by Coley toxin and bacillus Calmette-Guérin (BCG) in association with anticancer responses [36]. On the other hand, TLR signaling also induces inflammatory responses, thereby potentially favoring tumor growth as well [37]. An emerging notion is that, in addition to PAMPs, TLRs also recognize a wide range of self-derived molecules called as DAMPs released upon cellular stress that can alter TME [37, 38]. Thus, as described below, TLRs apparently show both pro-tumor and antitumor functions [37, 39]. This may be attributed to different tumor-inducing protocols or mice facilities, which can affect developmental process of tumor and commensal microbiota.
TLR1/2/6
These TLRs are expressed on the cell surface [34]. TLR2 forms heterodimers with TLR1 or TLR6 and recognizes a variety of PAMPs including lipopeptides, peptidoglycan, lipoteichoic acid, lipoarabinomannan, zymosan, glycosylphosphatidylinositol-anchored mucin-like glycoproteins from Trypanosoma cruzi, and hemagglutinin protein from bacteria or yeast [34]. TLR2 has also been shown to recognize endogenous ligands such as biglycan, hyaluronic acid, versican, and surfactant protein A [34]. The role of TLR2 in the regulation of oncogenesis has also been studied in animal models, with evidence that TLR2 signaling may play antitumor or pro-tumor function depending on model studied.
TLR2-deficient mice show exacerbated tumor growth in a model of hepatocellular carcinoma (HCC) in which a single injection of N-nitrosodiethylamine (DEN) is followed by repeated administration of carbon tetrachloride (CCl4) (hereafter called DEN/CCl4 model) [40]. It is suggested that an attenuated antitumor immune response including impaired IFN-γ expression, loss of cellular senescence, and autophagy are responsible for the expansion of tumor growth [40]. Similarly, a different study shows that TLR2-deficient mice develop more and larger intestinal tumors in the DEN/CCl4 model [41]. Tumor development is characterized by an increase in levels of IL-6, IL-17A, and signal transducer and activator of transcription 3 (STAT3) phosphorylation in the intestinal TME [41].
On the other hand, in a mouse gastric cancer model in which cancer cells display hyperactivation of STAT3, TLR2 deficiency results in reduced tumor burden, indicating a pro-tumor role of TLR2 [42]. This effect is independent of inflammation and is characterized by impaired proliferation and increased apoptosis of the gastric epithelial cells of the host [42]. These phenomena are explained by the suppression of phosphatidylinositol-3 kinase (PI3K), serine-threonine kinase 1 (AKT), extracellular signal-regulated kinase (ERK1/2), c-Jun N-terminal kinase (JNK), and NF-κB pathways in these cells due to the TLR2 deficiency [42]. In addition, TLR2 signaling may also play a role in maintenance of “stemness” in normal stem cells as well as gastric tumor cells [42]. TLR2-deficient mice also show slower metastatic growth of Lewis lung carcinoma (LLC) cells as compared to wild-type (WT) mice in a model of lung cancer [43]. Enhanced tumor growth in WT mice may be due to the activation of TLR2:TLR6 complex by LLC-derived versican, resulting in secretion of tumor necrosis factor-α (TNF-α) by myeloid cells which is known to promote tumor growth [43].
TLR3
TLR3 is localized within endosomes where it recognizes endocytosed double-stranded RNA (dsRNA), typically derived from viruses [34] as well as self-derived messenger RNA released from dead cells [34]. There is accumulating evidence to suggest that TLR3 functions to promote antitumor immunity. In an implanted transgenic adenocarcinoma model of prostate cancer, TLR3-deficient mice exhibit increased tumor growth as compared to controls [44]. In this model, TLR3-type I IFN signaling pathway enhances the activation of NK cells for antitumor responses [44]. Consistent with this, several studies have examined potential therapeutic effects of polyinosinic-polycytidylic acid (poly I:C), a synthetic TLR3 ligand, in the treatment of cancers. The proposed mechanisms of the poly I:C-TLR3 axis are (a) induction of IRF3-dependent NK-activating molecule (INAM) on DCs [45], (b) skewing of tumor-infiltrating macrophages toward a M1 phenotype [46], and (c) activation of DCs including the production of type I IFNs for enhancing effective cytotoxic T cell immune responses [47].
On the other hand, TLR3 has also been implicated in enhancing the growth of tumors in a spontaneous lung metastasis model in which lung metastasis is suppressed in TLR3-deficient mice [48]. It is further suggested that an RNA(s) derived from tumor exosomes activates TLR3 expressed in lung epithelial cells, leading to neutrophil recruitment and development of pre-metastatic niche, which results in the promotion of tumor progression [48].
As mentioned above, the TLR3 agonist poly I:C has been considered a promising adjuvant for cancer immunotherapy for several decades. Although effective, this therapy has been shown to cause life-threatening side effects, such as cytokinemia [36, 49, 50]. In this context, a recent study reported a new type of synthetic RNA that was designed to selectively activate the TRIF pathway, thereby effectively activating NK cells and cytotoxic T cells in tumor-loaded mice without inducing a severe cytokine storm induced by other types of dsRNA [51].
TLR4
TLR4 is expressed on the cell surface and recognizes lipopolysaccharide (LPS), a component of gram-negative bacteria [34]. It has also been reported that TLR4 recognizes various endogenous ligands, such as high-mobility group box protein 1 (HMGB1), heat shock proteins (HSPs), biglycan, hyaluronic acid fragments, and oxidized low-density lipoprotein [34].
In a mouse colon cancer model in which a single dose of azoxymethane (AOM) is combined with exposure to dextran sodium sulfate (DSS) (termed AOM/DSS model hereafter), TLR4-deficient mice show decreased tumor burden, suggesting its pro-tumor role [52, 53]. Mechanistically, it is proposed that TLR4 signaling (presumably activated by commensal bacteria) in colonic epithelial cells induces (a) immunosuppressive cyclooxygenase-2 (COX-2), (b) amphiregulin that activates epidermal growth factor receptor (EGFR) signaling [52], and (c) TME formation by the recruitment of COX-2-expressing macrophages [53]. In accordance with above results, mice carrying a transgene for a constitutively active TLR4 protein under the villin promoter in intestinal epithelial cells are more susceptible to tumor development in the same mouse model [54]. In another colon cancer model, however, TLR4 exhibits an antitumor effect. Mice harboring constitutively active TLR4 in the intestinal epithelial cells show decreased tumor burden in the APCmin/+ mouse model of spontaneous intestinal tumorigenesis (APCmin model) [55]. Tumor cells isolated from the intestine of these mice show elevated expression of IFN-β and caspase-3 activation, which correlate with increased apoptosis in vivo [55].
In a HCC model, i.e., the DEN/CCl4 model, diminished tumor development is observed in TLR4-deficient mice as compared to WT mice, implicating the pro-tumor function of TLR4 signaling [56]. Microbiota of the intestine and TLR4 on liver-resident cells are thought to mediate increased proliferation, preventing apoptosis in tumor cells and expression of epiregulin, a hepato-mitogen [56]. Similarly, a reduction in the development of HCC is observed in TLR4-deficient mice in DEN-induced HCC model [57]. One study, however, shows that TLR4-deficient mice exhibit higher tumor burden in DEN-induced HCC model [58]. This exacerbation of carcinogenesis is explained by impaired DNA repair and subsequent oxidative stress [58].
In a skin cancer model, which is induced by the combination of 7,12-dimethylbenz[a]anthracene (DMBA) and croton oil, TLR4-deficient mice also show resistance to carcinogenesis, indicating a tumor-promoting role of TLR4 signaling [59]. Mechanistically, it is argued that HMGB1, released from dying keratinocytes, activates TLR4 and enhances inflammation, thereby promoting tumor development [59]. In addition, in a genetically engineered mouse model of melanoma, i.e., the HGF-CDK4(R24C) mice, which harbor deregulated receptor tyrosine kinase signaling of hepatocyte growth factor (HGF) and impaired cell cycle control by an oncogenic CDK4(R24C) mutation, TLR4 deficiency abrogates the UV-induced enhancement of lung metastasis [60]. In this experimental setting, TLR4 is activated by extracellular HMGB1 released from UV-damaged keratinocytes causing the recruitment of neutrophils that induce angiogenesis and migration of melanoma cells toward endothelial cells [60]. On the other hand, another study indicates that TLR4-deficient mice exhibit enhanced tumor burden in the DMBA skin cancer model, and this is accompanied by elevated levels of serum IL-17 and decreased level of IFN-γ, suggesting impaired Th1-mediated antitumor responses [61].
Reports describing roles of TLR4 in other organs are rather limited. In the pancreas, mice lacking TLR4 in hematopoietic cell compartment show reduced lesions of pancreatic intraepithelial neoplasia in the p48Cre;KrasG12D pancreatic cancer model [62]. Contrary to this, TLR4 deficiency results in exacerbated tumor in the lung after injection of 3-methylcholanthrene and butylated hydroxytoluene (BHT) [63]. TLR4 has also been shown to be protective for both DMBA-induced mammary cancer model [64] and 4 T1-inoculated metastasis model [65]. The pro-tumor or antitumor outcome of TLR4 signaling may depend on the activation status of the MyD88 and TRIF signaling pathways which have the tendency to play pro-tumor and antitumor growth roles, respectively [50, 66].
TLR5
TLR5 is expressed on the cell surface [34] where it recognizes flagellin, a component of bacterial flagella [34]. One study reported that the ectopic expression of flagellin by tumor cells induced detectable antitumor immune response against EL4 murine lymphoma, thus utilizing flagellin as a tumor vaccine [67]. This effect is mediated by TLR5 signaling and inflammasome induced by the activation of nucleotide-binding oligomerization domain (NOD) containing-like receptor (NLR) [67]. However, a recent report demonstrated that TLR5 could enhance tumor growth. Here, TLR5-deficient mice show abrogated tumor growth in a genetically engineered mouse sarcoma model [68]. TLR5 deficiency is associated with decreased expression of IL-6, which leads to reduced recruitment of myeloid-derived suppressor cells (MDSCs), which cause γδ-T cells to release galectin-1, which suppresses antitumor adaptive immune responses and accelerates tumor progression. Interestingly, TLR5-dependent acceleration of the tumor growth is mediated through interactions with commensal microbiota [68].
TLR7/8
TLR7 recognizes single-stranded RNA (ssRNA), typically derived from RNA viruses, within endosomes [34]. It is highly expressed on plasmacytoid DCs (pDCs) and is crucial for the massive release of type I IFNs against RNA viruses that define this cell type [34]. Human TLR8 also recognizes viral ssRNA [34]. However, TLR8-deficient mouse cells show no defects in cytokine production against viral ssRNA [34]. TLR7 can also recognize self-derived ssRNA bound to autoantibodies [34].
Small molecule agonists of TLR7/8 have been proposed as antitumor immunotherapy drugs. Imiquimod has been known to activate TLR7-MyD88 signaling to exert antitumor effect [69, 70]. Several studies have elucidated detailed mechanisms of antitumor function of this compound. For example, imiquimod makes DCs produce type I IFNs and transform themselves into cells which can directly eliminate tumor cells via TNF-related apoptosis-inducing ligand (TRAIL) and granzyme B activities [71, 72]. In addition, immunostimulatory RNA oligonucleotides with specific sequence also induce antitumor immune response through NK cells in a TLR7-dependent manner [73].
Genetic studies, however, show an opposite role of TLR7 on tumor development. One study reveals that TLR7 deficiency in hematopoietic cells abrogates tumor development in a mouse pancreatic cancer model in which a mutated K-Ras gene is expressed [74]. Another study shows that TLR7-deficient mice are associated with less tumor burden and prolonged survival in LLC lung cancer metastasis model [75]. There is no clear explanation for the seemingly discrepant results between studies employing synthetic ligands and genetic studies in terms of effects of TLR7 signaling on tumor progression; it may depend on the magnitude and duration of TLR7 activation between synthetic and endogenous ligands, and this may also be the case for other PRRs (see below).
TLR9
The role of TLR9 signaling in antitumor immunity has been underscored by numerous reports. Most notably, a therapeutic effect of CpG-oligodeoxynucleotide (CpG-ODN), a TLR9 ligand, on tumor growth has been extensively studied [76–78]. CpG-ODN treatment induces a significant antitumor effect in C3 murine model of cervical cancer and C26 murine colon cancer model, wherein the tumor regressions and extended survival resulting from this therapy requires the participation of CD8+ T cells [76, 77]. CpG-ODN also has a suppressive effect on a murine neuroblastoma cell line neuro2a, which seems to be mediated by NK cells [78]. Furthermore, combination of another TLR9-stimulating ODN with trastuzumab, a monoclonal antibody against human epidermal growth factor receptor 2 (HER2), is efficient for the control of trastuzumab-resistant human breast cancer cells in a mouse xenograft model [79]. Interestingly, this ODN modulates the interaction of TLR9 with HER receptors at the membrane level, thereby inhibiting the HER-dependent growth signal [79].
On the other hand, TLR9 may promote tumor growth. Orthotopically implanted pancreatic cancer cells carrying mutated genes for K-Ras and p53 show delayed growth in TLR9-deficient mice as compared with WT mice [80]. This effect is specific to the pancreatic TME, as pancreatic cells implanted subcutaneously do not show reduced growth in TLR9-deficient mice [80]. Mechanistically, TLR9 activation of pancreatic stellate cells (PSCs) results in chemokine (C-C motif) ligand 11 (CCL11) production, leading to tumor cell proliferation via its receptor chemokine (C-C motif) receptor 3 (CCR3). Moreover, it is shown that PSCs can recruit regulatory T cells (Tregs) into the peritumoral site in a TLR9-dependent manner, possibly through the CCL3-CCR5 axis. Additionally, TLR9 in immune cells is also responsible for MDSC recruitment to the TME, further exacerbating tumor progression [80].
25.1.2 Role of Cytosolic Nucleic Acid Sensors in Tumor Development
Cytosolic nucleic acid-sensing PRRs are expressed in almost all cell types and detect RNA and DNA or their mimetics to evoke innate immune responses [81]. The induction of type I IFNs is the hallmark of the activation of these cytosolic PRRs, and this induction underlies effective antiviral responses through the PRR’s recognition of virus-derived nucleic acids [81]. Since the antitumor function of type I IFNs has been well appreciated, the role of these PRRs in antitumor immunity has also been the focus of attention [82].
RLRs
RIG-I (also known as DDX58) and melanoma differentiation-associated 5 (MDA5) sense dsRNA, a replication intermediate for RNA viruses, leading to the robust production of type I IFNs in infected cells [83]. Another member of this family, laboratory of genetics and physiology 2 (LGP2) acts as a negative feedback regulator of RIG-I and MDA-5 [84], but it may promote the MDA5 signaling pathway in some cases [85, 86]. Despite the overall structural similarity between these two activatory PRRs, they detect distinct viral species, because of different structural features of the virus-derived RNAs [83]. RIG-I binds specifically to ssRNA containing 5′-triphosphate such as viral RNA and in vitro-transcribed dsRNA [87, 88]. It has also been shown that RIG-I binds preferentially to short dsRNA, while MDA5 preferentially recognizes long dsRNA [89]. Both RLRs share in common signaling features. Upon recognition of dsRNA, they are recruited by the adaptor MAVS (also known as IPS-1, CARDIF, or VISA) to the outer membrane of the mitochondria leading to the activation of several transcription factors including IRF3, IRF7, and NF-κB [81]. While IRF3 and IRF7 primarily mediate the induction of type I IFNs, NF-κB regulates the gene induction for inflammatory cytokines (for further details, see [90–92]).
That the activation of these RLRs results in the robust induction of type I IFNs prompted many investigators to assess their role in antitumor responses, as type I IFNs modulate a plethora of important cellular functions other than antiviral responses including effects on cell growth, differentiation, and antitumor immunity [82]. In humans, low RIG-I expression in HCC tissue predicts a poorer prognosis and a higher resistance to IFN-α therapy [93]. The tumor-suppressive role of RIG-I has been validated in RIG-I-deficient mice in the model of HCC [93]. It has also been reported that RIG-I activation induces the secretion of extracellular vesicles (EVs) from melanoma cells, which exhibit expression of the NKp30-ligands on their surface, thus triggering NK cell-mediated elimination of melanoma cells [94]. Since MDA5 triggers similar if not identical signaling pathways as RIG-I, there is the direct implication that MDA5 is also involved in tumor development [83]. Since LGP2 enhances the survival and activation of CD8+ T cells [95], it is also possible that LGP2 plays a direct regulatory role in antitumor immune responses.
The fact that the RIG-I activation also induces intrinsic apoptosis through BH3 family, which occurs independently of type I IFN signaling, led to the study of exploiting RIG-I-mediated apoptosis to selectively eliminate malignant cells [96]. As such, much effort has been devoted to the development of targeting drugs of the RLR signaling pathway for cancer therapy with the working hypothesis that the induction of type I IFN, apoptotic, or both pathways may play a crucial role(s) [97–102].
DNA Sensors
Among DNA-sensing PRRs reported thus far, the cyclic GMP-AMP (cGAMP) synthase (cGAS) is one of the best characterized molecules for its role in antiviral immunity. Viral DNA released into the cytosol is catalyzed by cGAS and converted to cGAMP which in turn binds to stimulator of IFN genes (STING) to activate its downstream signaling pathways including type I IFNs [103]. Perhaps expectedly, some studies have implicated the involvement of cGAS in the host immune responses against tumors [104–106]. cGAS-expressing macrophages are essential for IFN-β production in response to tumor-derived DNA [104]. Consistent with this, DCs are stimulated by irradiated tumor cells to release IFN-β, and this promotes antigen-specific CD8+ T cell activation in cGAS-dependent manner [105]. In addition, cGAS is also required for IFN-β production triggered by the treatment with anticancer drugs, cisplatin and camptothecin [106].
As well as cGAS-mediated pathway, other DNA-sensing mechanisms are also important for the response to cytosolic nucleic acids. DNA-dependent activator of IRFs (DAI) associates with TBK1 and IRF3 and responds to cytosolic DNA for type I IFN induction [107], and its antitumor function has been reported [108]. Another DNA-sensing PRR, human IFN-γ-inducible protein 16 (IFI16), and its mouse ortholog p206 induce IRF3 activation and IFN-β production upon cytosolic DNA stimulation [109], wherein recruitment of STING to IFI16 may be a critical step in the signaling pathway [109]. A similar association between STING is also observed with another PRR, DDX41 [110]. DDX41 recognizes various DNA and functions as a direct sensor for cyclic-di-GMP and cyclic-di-AMP, which are second messengers for STING activation as cGAMP [111]. The tumor-suppressive role of DDX41 has been reported in hematopoietic neoplasia caused by mutations in DDX41 gene [112]. Furthermore, it is implicated that some DNA damage-responding molecules, such as DNA-dependent protein kinase (DNA-PK) and meiotic recombination 11 (MRE11), are involved in cytosolic DNA sensing [113, 114]; however, whether these DNA sensors regulate tumor development is largely unknown. Overall, as tumor-derived DNA is taken up by antigen-presenting cells in TME and activates DNA-sensing PRRs, their antitumor functions have been the focus of much attention in basic and clinical cancer immunology [104].
The role of STING in antitumor immune response has been the particular focus of attention [103]. In the mice inoculated with tumor cells expressing an immunogenic peptide, STING appears to contribute to antitumor response to these cells, wherein tumor-derived DNAs, which are taken up by antigen-presenting cells in TME, stimulate STING and induce IRF3-mediated IFN-β production for the expansion of antigen-specific CD8+ T cells [104]. Similarly, STING-dependent IFN-β production and CD8+ T cell activation are triggered in irradiation-treated tumor [105]. It is suggested that such IFN-β upregulation is induced by dead cell-derived DNA via IRF3 activation [115]. Furthermore, STING promotes IL-18 and IL-22BP expression in tumor tissue and suppresses AOM/DSS colon carcinogenesis [116]. STING signaling, on the other hand, has been reported to downregulate the expression of pro-inflammatory IL-6 that activates the pro-tumor transcription factor STAT3 [117]. A study using a glioma model shows that STING ameliorates the associated cancer burden with enhanced CD8+ T cell activation and reduced infiltration of immunosuppressive cells such as MDSCs and Tregs in the brain [118].
In general, the targeting of STING for its activation has been beneficial for the treatment of cancer in many mouse models. Administration of cGAMP decreases tumor growth of colon 26 cells in association with DC maturation [119]. Moreover, cGAMP also retards B16 melanoma cell growth in vivo with the activation of CD8+ T cells in TME through type I IFN signaling [120].
The activation of STING by a chemical compound ML RR-S2 can exert antitumor activity in several mouse models [121]. ML RR-S2 is also experimentally useful as an adjuvant for anticancer vaccine with GM-CSF-expressing dead tumor cells [103]. This therapeutic strategy, called as STINGVAX, has been shown to be effective against B16 mouse melanoma tumor that are resistant to a checkpoint therapy [103]. Related to this, it is also noteworthy in this context that some anticancer chemical drugs are also DNA adduct-forming agents that trigger cell death and release STING agonists [122]. It has been discovered that 5,6-dimethylxanthenone-4-acetic acid (DMXAA), a vascular-disrupting agent, directly binds to STING and it is now used as a STING agonist for cancer therapy [123]. DMXAA induces IFN-β production from antigen-presenting cells and shows potent therapeutic activity against in vivo growth of tumor cells through enhancement of adaptive immunity [121]. In addition, macrophages are targeted by DMXAA and polarized from an M2-type to M1-type [124, 125]. Furthermore, DMXAA treatment promotes immunologic memory against tumor [121], and mice that rejected tumors by DMXAA administration are resistant to secondary-challenged tumor. Notably, intratumoral administration of this drug inhibits the tumor growth developed at other distant site [121]. As such, the STING-activating molecules, such as cytosolic cyclic dinucleotides and chemical compounds, may pave the way(s) for the establishment of effective cancer immunotherapy.
On the other hand, there are reports showing a pro-tumor role of STING [106, 126]. DNA released into cytosol in carcinogen-damaged cells stimulates STING to induce inflammatory cytokine expression and exacerbates DMBA-induced skin carcinogenesis [106]. It has also been reported that intradermal growth and lung metastasis of LLC tumor cells are enhanced by STING in immunosuppressive host [126]. This study also shows that antitumor responses are induced against gp100-transduced LLC cells in STING-dependent manner, implicating that STING-mediated antitumor or pro-tumor response is dependent on the immunogenicity of tumor cells.
25.1.3 Role of CLRs in Tumor Development
CLR family members are primarily characterized by their detection of carbohydrates on bacteria, fungi, and viruses [127], while some CLRs can also recognize oxidized lipids and other DAMP molecules exposed by damaged cells [128, 129]. CLR activation leads to immunoreceptor tyrosine-based activation motif (ITAM)/immunoreceptor tyrosine-based inhibition motif (ITIM)-dependent or ITIM-independent signal transduction to induce host immune responses [129]. Some ITAM-based CLRs such as Dectin-1 and C-type lectin domain family 2 (CLEC-2) possess hemITAM motif and recruit spleen tyrosine kinase (Syk) to activate NF-κB via caspase activation and recruitment domain 9 (CARD9) [129]. Syk further transduces MAPK and nuclear factor of activated T cell (NFAT) pathways and induces reactive oxygen species (ROS) production which contributes to NACHT, LRR, and PYD domain-containing protein 3 (NALP3) activation [129]. Other ITAM-based CLRs, represented by Dectin-2, Mincle, and macrophage C-type lectin (MCL), associate with ITAM-containing adaptor protein such as Fc receptor γ (FcRγ) chain, leading to Syk-dependent signal transduction [129]. ITIM-containing CLRs inhibit the activation of NF-κB and STAT5 as well as ITAM-based signaling pathway through Src-homology 2 domain-containing phosphatase-1 (SHP-1) and SHP-2 [129]. In addition to signal transduction, CLRs also drive the phagocytotic system of myeloid cells to promote the uptake of invading pathogens and abnormal self-derived molecules [130]. Through these mechanisms, CLRs play critical roles in regulating innate and adaptive immune systems.
CLR involvement in tumor development has not been intensely analyzed. Some recent studies have revealed that CLRs control tumor growth and metastasis, functioning as key innate receptors to trigger both anti- and pro-tumor host responses.
Dectin-1
Dectin-1 mainly recognizes β-glucan structures and contributes to the defense against bacterial and fungal infection [127]. Activation of Dectin-1 with such carbohydrates leads to Syk-dependent signal transduction through hemITAM motif on itself [129]. In the control of subcutaneous tumor growth and lung metastasis, Dectin-1 induces antitumor responses through the enhancement of NK cell cytotoxicity against tumor cells [27]. This antitumor mechanism is triggered by Dectin-1 recognition to N-glycan structures on tumor cells [27]. Although Dectin-1 is a β-glucan receptor, a variety of glycan structures on cancer cells likely also bind to Dectin-1 [27]. Cancer cells activate Dectin-1 signaling in myeloid cells and promote antitumor killing of NK cells in cell-to-cell contact-dependent manner [27]. Supporting this, the expression of INAM, a membrane protein to drive NK cell activation, is upregulated by Dectin-1 in the presence of cancer cells [27]. NK cell-mediated tumor killing can also be induced against liver-metastasizing cancer cells. Dectin-1 enhances cytotoxic activity against SL4 colon cancer cells by NK cells to suppress liver metastasis [28].
Dectin-1-dependent antitumor immune responses can be useful for cancer therapy. The administration of lentinan, a purified β-glucan isolated from shiitake mushroom, suppresses gastric cancer development in human study [131]. In addition, sizofiran, Schizophyllum commune-derived β-glucan, improves the prognosis of ovarian cancer patients when combined with cisplatin, adriamycin, and cyclophosphamide [132]. The therapeutic effect of β-glucans has been also reported using murine in vivo models of tumor growth and for breast and liver metastasis [133]. The mechanisms of β-glucan-induced antitumor response have been intensely studied. Dectin-1 agonist curdlan activates DCs to elicit potent CD8+ T cell responses and markedly reduces lung metastasis of B16 melanoma cells [134]. Moreover, β-glucan-containing extracts from a mushroom, which promotes the expansion of NK cells and upregulates the expressions of antitumor cytokines such as IFN-γ and IL-12, decrease tumor burden of colon cancer 26 cell-inoculated mice [135].
In addition to instigating antitumor immunity, β-glucans can inhibit pro-tumor host responses. Whole β-glucan particles (WGP) from Saccharomyces cerevisiae downregulate the immunosuppressive activity of MDSCs and dampen the expansion of MDSCs and Tregs in the TME [136]. WGP also converts immunosuppressive M2-like macrophages to M1-like cells in a Dectin-1 and CARD9-dependent manner [137]. CARD9 is a signaling molecule activated by ITAM-associated CLRs like Dectin-1 that can induce antitumor responses [129]. Mice treated with mushroom-derived β-glucans also show loss of immunosuppressive TME [138].
Dectin-2
Dectin-2 binds to mannose-rich carbohydrates to transduce Syk-dependent signaling [127]. Unlike Dectin-1, Dectin-2 does not possess an ITAM motif [129]. Instead, it associates with FcRγ chain which itself possesses ITAM motif to activate downstream pathways [129]. The role of Dectin-2 in tumor immunity is relatively unique as compared to other CLRs. Although Dectin-1 inhibits subcutaneous tumor growth and lung metastasis [27], Dectin-2 is not involved in these responses [28]. Notably, however, Dectin-2 contributes to the suppression of liver metastasis [28]. The underlying mechanism is unique in that Dectin-2 promotes phagocytosis of Kupffer cells, liver-residing macrophage, against cancer cells in vitro, although such Dectin-2-dependent uptake of cancer cells is not observed in bone marrow-derived macrophages and alveolar macrophages [28]. Consistent with this, tumor metastasis to liver is notably enhanced in Dectin-2-deficient mice [28]. Interestingly, the expression of IL-6 and CXCL1, which is induced when Dectin-2 is activated by fungi, remained unaffected when Dectin-2-deficient Kupffer cells interact with cancer cells [28]. This observation suggests that antitumor Dectin-2 signaling pathway(s) in Kupffer cells is distinct from the conventional one for antimicrobial responses.
Mincle
Macrophage-inducible C-type lectin (Mincle) binds mannose and trehalose-6,6′-dimycolate (TDM), a mycobacterial glycolipid [129]. Mincle also recognizes endogenous ribonucleoprotein spliceosome-associated protein 130 (SAP-130), which is released from dying cells [139]. Stimulation of Mincle with its ligand induces Syk-dependent signaling pathway through ITAM-possessing FcRγ chain [129]. In pancreatic oncogenesis of p48Cre; KrasG12D mice, Mincle establishes an immunosuppressive TME and promotes tumor development [140]. Mincle signaling enhances the production of IL-10 from T cells and the infiltration of MDSCs and M2-like macrophages in tumor [140]. This oncogenic process is associated with necroptosis and the induction of SAP-130 expression in pancreas [140]. Since SAP-130 administration into pancreas aggravates tumor growth, the ligation of Mincle with dead cell-released SAP-130 promotes oncogenic process with immunosuppression [140]. Interestingly, in spite of its relative importance in pancreatic tumor progression, Mincle doesn’t control liver metastasis of SL4 colon carcinoma cells [28].
CLEC-2
CLEC-2 is a receptor for a snake venom toxin, rhodocytin [127], and stimulation with rhodocytin induces similar signaling pathways to ITAM-dependent ones [129]. CLEC-2 also recognizes podoplanin, a mucin expressed on some cancer cells [141]. Although the study of CLEC-2 in vivo is challenged by difficulties of embryonic lethality of CLEC-2-deficient mice [142], the CLEC-2-podoplanin interaction is thought to be a key event in promoting cancer metastasis [141]. Platelets expressing CLEC-2 are activated by podoplanin on cancer cells triggering their aggregation [143]. The platelet aggregation supports the evasion of cancer cells from immune system essentially by masking their detection while also promoting metastasis by facilitating adhesion to the endothelial wall [144]. Furthermore, CLEC-2 activation induces the secretion of growth factors, chemokines, matrix proteins, and angiogenic factors, which can promote metastasis [141]. In addition, CLEC-2 also activates podoplanin signaling in cancer cells, which leads to the downregulation of E-cadherin and promotes metastasis [145, 146]. Furthermore, the interaction of podoplanin with platelet accelerates subcutaneous tumor growth of podoplanin-expressing PC-10 lung cancer cells [147]. As such, it is strongly suggested that CLEC-2 plays an important role in multiple steps of tumor development.
DC-SIGN
DC-specific ICAM-3 grabbing non-integrin (DC-SIGN) shows high affinity for mannose, fucose, N-acetylglucosamine, and Lewis antigens [127, 148]. DC-SIGN activation drives ITAM/ITIM-independent signaling through lymphocyte-specific protein 1 (LSP1) [129] and promotes cross-presentation of antigens to CD8+ T cells [149]. Consistent with this, DCs treated with Lewis X oligosaccharides-heparanase complex enhance CD8+ T cell response and possess the ability to reduce tumor growth of G422 glioblastoma cells in vivo [150].
On the other hand, DC-SIGN plays pro-tumor roles in some cases. DC-SIGN recognizes Lewis carbohydrates on carcinoembryonic antigen (CEA) and Mac-2-binding protein (Mac-2BP) which are mainly expressed by colon cancer cells [151–153]. Since ligation of DC-SIGN on macrophages to cancer cells induces IL-10 production [154], DC-SIGN likely helps to establish immunosuppressive TME. This notion is further supported by a report showing that DC-SIGN-deficient mice exhibits slower subcutaneous tumor growth of LLC cells compared to WT mice [155].
MGL
Macrophage galactose C-type lectin (MGL) has specificities to galactose and N-acetylgalactosamine for its ligand recognition [127, 129]. MGL binding to such carbohydrate moieties on microbes promotes their uptake and leads to antigen presentation [129]. Further, MGL recognizes carbohydrate antigens, Tn and sialyl Tn, on mucin 1 (MUC1) which are expressed on a variety of cancer cells [156, 157]. While MGL activation triggered by sialyl Tn-associated MUC1 enhances DC maturation and migration [158], anti-MGL blocking antibody inhibits the dissemination of foot pad-inoculated cancer cells to lymph nodes [159]. Therefore, MGL may have a pro-tumor role in lymph node metastasis.
MR
Mannose receptor (MR) recognizes mannose and promotes its endocytosis [127]. It has also been known that ovalbumin (OVA) conjugated with synthetic MR ligands, 3-sulfo-Lewis A and tri-N-acetylglucosamine, primes DCs to induce antigen-specific CD8+ T cell proliferation and Th1 polarization of CD4+ T cells [160]. Therefore, MR ligation with its ligand likely induces antitumor immune responses. Indeed, treatment by OVA conjugated with anti-MR antibody instigates CD8+ T cell responses in vivo and, together with CpG treatment, markedly reduces subcutaneous tumor growth of OVA-expressing B16 cells in MR-transgenic mice [161].
Nevertheless, MR also possesses pro-tumor activity. MR is involved in the recognition of tumor-specific mucin CA-125 and TAG-72 [162]. Stimulation of MR on tumor-associated macrophages (TAMs) with these molecules induces IL-10 production and inhibits IL-12 expression [162]. Moreover, MR expressed on tumor-activated liver sinusoidal endothelial cells (LSECs) inhibits antitumor cytotoxicity of liver sinusoidal lymphocytes (LSLs) [163]. MR further decreases and increases IFN-γ and IL-10 production, respectively, from LSLs of tumor-bearing mice [163]. These reports evoke the notion that MR promotes cancer development.
MCL
MCL is an FcRγ-associated receptor for TDM and a heterodimeric counterpart of Dectin-2 [164, 165]. Consistent with this and like Dectin-2, MCL enhances the phagocytotic activity of Kupffer cells and suppresses liver metastasis of SL4 colon carcinoma cells [28]. Interestingly, although MCL induces Mincle expression in bone marrow-derived macrophage [166], Mincle is not involved in the control of liver metastasis, as described above [28].
Other CLRs
The roles of other CLRs in cancer have been studied, albeit not extensively. Some studies indicate that DAMPs released in TME can regulate immune responses to cancer [167, 168]. Additionally, some DAMPs act as a ligand for CLRs. DC NK lectin group receptor-1 (DNGR-1) recognizes F-actin and actin complex with spectrin β and α-actinin, both of which are exposed by damaged cells [169, 170]. Furthermore, F-actin can drive Syk-dependent signaling in DNGR-1-expressing cells [169], implicating its involvement of DNGR-1 in host immune responses to tumor. In addition, CLEC12A binds to uric acid crystals from dead cells and inhibits neutrophil-associated inflammation in vivo after the challenges with necrotic cells or irradiation [171]. Uric acid also contributes to DC maturation, CD8+ T cell priming, and Th17 differentiation [172–174]. Therefore, CLEC12A may regulate tumor development in dead cell-enriched microenvironment.
CLRs might have roles beyond sensing DAMPs in the regulation of tumor immunity. Mannose-binding lectin (MBL) recognizes Lewis antigens on SW1116 human colorectal carcinoma cells [175, 176]. Moreover, blood dendritic cell antigen 2 (BDCA-2), which is exclusively expressed on pDC and activated by asialo-oligosaccharides with terminal galactose to suppress type I IFN production, binds to several kinds of human cancer cells such as ovarian and colon carcinoma cells [177]. ITAM-based CLRs other than those described above, for example, SIGN-related gene 3 (SIGNR3) and myeloid DAP12-associating lectin (MDL-1), can also be involved in tumor growth, since CARD9 promotes colon tumorigenesis in males of APCmin mice [178]. Further analysis for the functions of these receptors in immune response to cancer promotes a better understanding of CLR-mediated regulation of tumor development. In line with this, how ITIM-associated CLRs are involved in the regulation of tumor growth and metastasis, which is obscure so far, is an intriguing question to be clarified.
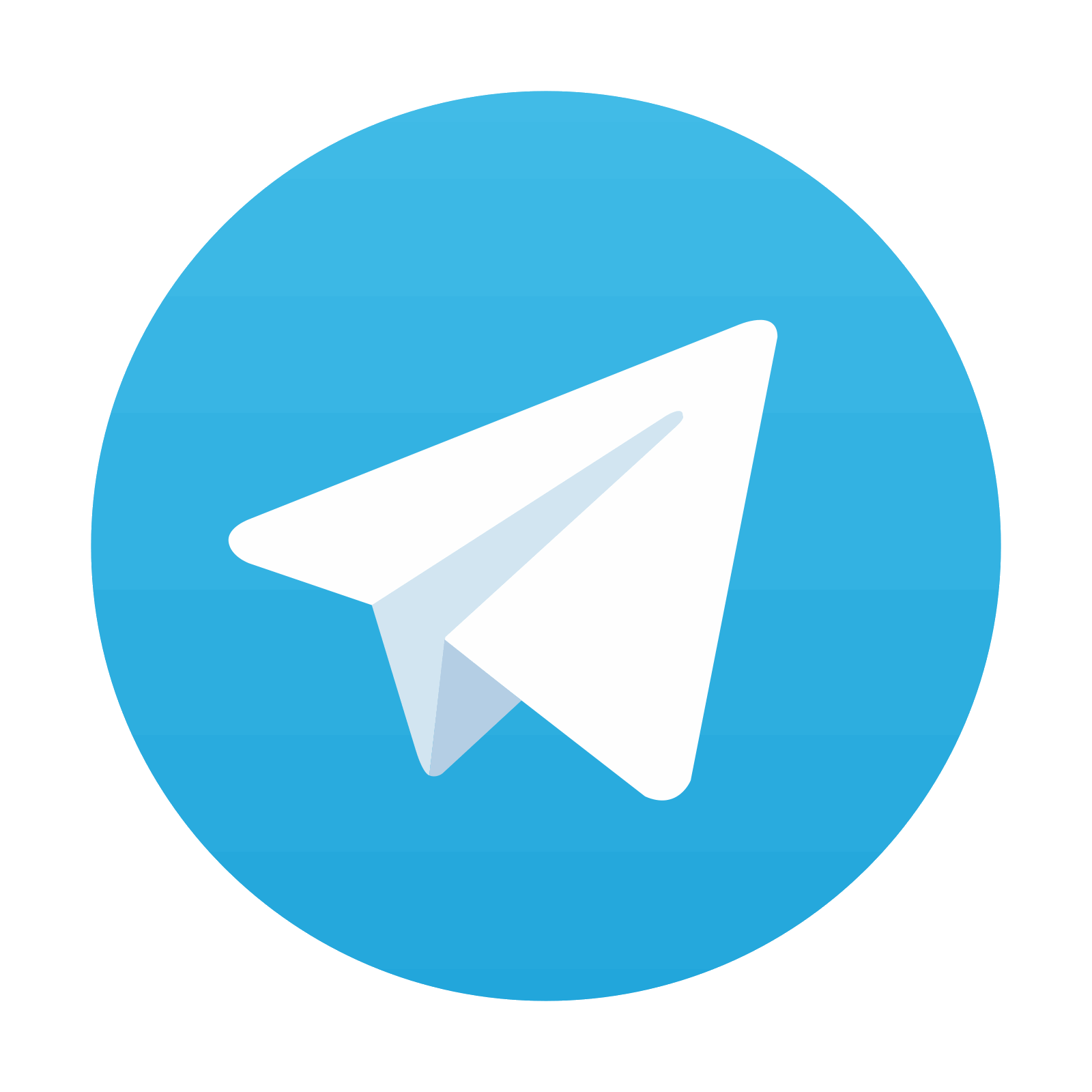
Stay updated, free articles. Join our Telegram channel
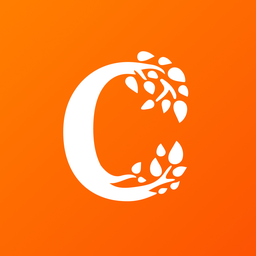
Full access? Get Clinical Tree
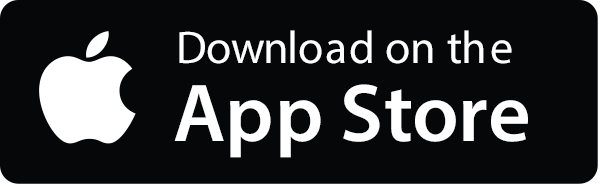
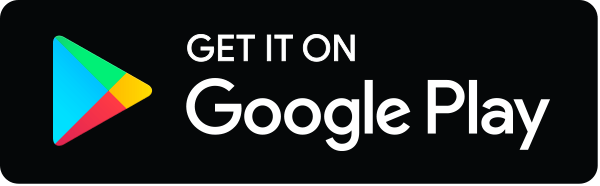