Chapter Outline
HEREDITARY THROMBOTIC DISORDERS
INHERITED ABNORMALITIES OF DIMINISHED FIBRINOLYSIS
MANAGEMENT OF PATIENTS WITH INHERITED THROMBOTIC DISORDERS
Acute Thrombosis and Long-Term Management
Coumarin-Induced Skin Necrosis
Homozygous or Doubly Heterozygous Protein C Deficiency and Neonatal Purpura Fulminans
Evaluation of Relatives of Patients with Hereditary Thrombophilia
For many years, researchers suspected that hereditary coagulation defects underlie a large percentage of venous thromboembolic events that could not be attributed to identifiable acquired risk factors. Acquired risk factors for venous thrombosis consist of a heterogeneous group of conditions and clinical disorders with diverse and poorly understood prothrombotic mechanisms. With expanding scientific knowledge of the hemostatic mechanism and the regulatory role of natural anticoagulants, major advances have been made in identifying hereditary defects in the heparan sulfate–antithrombin and protein C pathways during the past three decades. To date, whole genome screening and sequencing efforts have identified several additional genes of interest, although each individually makes a relatively small contribution to thrombotic risk. In this chapter we review the hereditary disorders of thrombosis and fibrinolysis associated with an increased risk for thrombosis. Issues relating to diagnosis and treatment of the hereditary thrombophilias are also discussed.
Hereditary Thrombotic Disorders
Before 1980, the medical literature included only isolated reports of thrombosis in the pediatric population. Although, when compared with adults, thromboembolic events are uncommon in young patients, the incidence is increasing rapidly. This increase is particularly true of children who experience stays in the hospital and is attributed to advances in critical care medicine, improved survival of high-risk patients (e.g., neonates and children with severe congenital heart disease), and increased awareness. The paucity of thrombotic events in children may be related more to vascular/endothelial factors than to hematologic factors, although some of the physiologic inhibitors do not attain full normal adult levels until mid childhood (e.g., protein C and protein S; see Chapter 5 ). Even patients with hereditary deficiencies of antithrombin (AT), protein C, or protein S, in association with moderate reductions in the levels of these natural physiologic anticoagulants, have few thrombotic symptoms during early childhood. During the past 30 years, however, more vigorous supportive and specific treatment measures for sick preterm infants have resulted in a markedly improved survival rate of even very preterm infants. Because these infants are quite prone to thrombotic events, the physiologic “hypercoagulability” of the developing hemostatic system has received increased attention and is discussed elsewhere (see Chapter 5 ). Thus the newborn infant can be expected to be at greater risk for thrombosis if he or she also has an underlying hereditary deficiency of an anticoagulant protein. Inherited alterations of coagulation proteins that have been clearly associated with a prethrombotic state are listed in Box 33-1 .
- •
Antithrombin deficiency
- •
Protein C deficiency
- •
Protein S deficiency
- •
Resistance to activated protein C because of the factor V Leiden mutation (factor V Arg506Gln)
- •
Prothrombin G20210A mutation
- •
Dysfibrinogenemias (rare)
Antithrombin Deficiency
AT is a plasma protein synthesized by the liver that binds and neutralizes the serine proteases generated by the coagulation cascade, including thrombin, factor Xa, and factor IXa (see Chapter 27 ). Inhibition of these coagulation serine enzymes by AT is relatively slow but is markedly enhanced by binding to heparin. AT has two major active functional sites: the reactive center toward the carboxyl end and the heparin-binding site located at the amino terminus of the molecule. Thrombin cleaves the reactive site followed by the formation of an inactive complex, which is rapidly cleared from the circulation.
AT deficiency was the first hereditary hypercoagulable disorder identified. In 1965, Egeberg investigated a Norwegian family with a strong history of thrombosis and found that affected persons had plasma AT concentrations that were 40% to 50% that of normal concentrations. Subsequent studies described families with similar clinical and laboratory abnormalities. Heterozygosity for AT deficiency can be found in approximately 4% of families with inherited thrombophilia and in 1% of consecutive patients with a first episode of deep venous thrombosis.
The thrombotic risk associated with AT deficiency depends on the population selected. In older reviews of AT deficiency that included families with a high penetrance of thrombosis, more than 50% of affected patients experienced venous thrombotic episodes. The initial clinical manifestations occur spontaneously in about 42% of subjects but are related to pregnancy, parturition, hormonal contraceptives, surgery, or trauma in 58% of patients. In children, the odds ratio (OR) of thrombosis with AT deficiency is estimated to be 9.44 (95% confidence interval [CI], 3.34 to 26.66). The most common sites of disease are the deep veins of the leg and the mesenteric veins. Recurrent thrombotic episodes occur in approximately 60% of affected persons, and clinical signs of pulmonary embolism are evident in 40% of affected persons. The literature includes case reports of neonatal and childhood arterial ischemic stroke in association with AT deficiency, but a causal relationship remains to be proven. Although cases have been reported in which AT-deficient infants sustained cerebral venous thrombosis, thrombotic episodes are relatively uncommon in affected children before puberty, after which the risk of thrombosis increases substantially with advancing age. First-degree relatives of symptomatic persons with AT deficiency have an eightfold to tenfold increased risk for thrombosis compared with the risk in noncarriers. In the Leiden Thrombophilia Study—a case-control study of 474 consecutive patients after an initial episode of deep venous thrombosis —the prevalence of AT deficiency was just 1.1%, and the OR for thrombosis was 5.0.
AT deficiency is inherited in an autosomal-dominant fashion and thus affects both sexes equally. Two major types of inherited AT deficiency have been delineated ( Table 33-1 ). The type I deficiency state is a result of reduced synthesis of biologically normal protease inhibitor molecules. In these cases the antigenic and functional activities of AT in blood are reduced in parallel. The molecular basis of this disorder is either deletion of a major segment of the AT gene or, more commonly, the occurrence of small deletions/insertions or single base substitutions. These mutations introduce a frameshift, a direct termination codon, a change in messenger ribonucleic acid (mRNA) processing, or unstable translation products. The AT mutation database includes more than 100 distinct mutations in patients with type I deficiency.
Type | Antigen | Activity | Heparin Cofactor | Progressive Antithrombin |
---|---|---|---|---|
I | Low | Low | Low | |
II | Active-site defect | Normal | Low | Low |
Heparin-binding site defect | Normal | Low | Normal |
The second type of AT deficiency is produced by a discrete molecular defect within the protease inhibitor (type II). Plasma levels of AT are greatly reduced as judged by functional activity, whereas AT antigen is essentially normal. The first family with type II AT deficiency was described in 1974. As additional families with this type II AT deficiency have been reported, they have been further subcategorized on the basis of two different functional assays of AT activity. The first is the antithrombin-heparin cofactor assay, which measures the ability of heparin to bind to lysyl residues on the inhibitor and catalyze the neutralization of coagulation enzymes such as thrombin and factor Xa. The second test is the progressive AT activity assay, which quantitates the capacity of this inhibitor to neutralize the enzymatic activity of thrombin in the absence of heparin.
Heterozygous type II patients with both diminished heparin cofactor activity and progressive AT activity have mutations near the thrombin-binding site at the carboxyl terminus of the molecule; patients with reductions only in heparin cofactor activity have mutations in the heparin-binding domain at the amino terminus of the molecule. The distinction between type II defects is clinically relevant because variants with heparin-binding site defects are infrequently associated with thrombotic events, except when they are present in the homozygous state. Patients with homozygous mutations in the heparin binding site leading to AT deficiency were young children in whom severe venous or arterial thrombosis (or both) developed along with plasma antithrombin-heparin cofactor levels measuring less than 10%. In each instance, a history of parental consanguinity was noted, and the parents had type II AT deficiency with heparin-binding site defects.
The prevalence of type I AT deficiency in the adult population is approximately 1 in 2000. Studies of healthy blood donors with functional assays that measure heparin cofactor activity have found a prevalence of AT deficiency in the general population of 1 in 250 to 1 in 500 ; a substantial number, however, have a type II defect with mutations at the heparin-binding site. The best single screening test for the disorder is the antithrombin-heparin cofactor assay, which measures inhibition of factor Xa; it is the functional AT assay that is most widely used today.
The mean concentration of AT in normal pooled plasma is approximately 140 µg/mL. Most laboratories report a normal range between 75% and 120% of normal pooled plasma for antithrombin-heparin cofactor determinations and a somewhat wider range for immunoassay results. A variety of pathophysiologic conditions can reduce the concentration of AT in blood. AT levels can drop substantially in patients with disseminated intravascular coagulation (DIC), sepsis, burns, and severe trauma but less commonly in the setting of acute thrombosis. Reduced levels can also be seen in persons with liver disease as a result of decreased synthesis or in persons with nephrotic syndrome as a result of urinary protein loss. Modest reductions are also found in users of oral contraceptives or estrogens. In addition, the administration of heparin decreases plasma AT levels, presumably by accelerated clearance of the heparin-AT complex. Thus evaluation of plasma samples from persons during a period of heparinization can potentially lead to an erroneous diagnosis of AT deficiency.
Healthy newborns have about half the normal adult concentration of AT and gradually reach the adult level by 6 months of age. Levels may be considerably lower in infants born at 30 to 36 weeks of gestation and are even further reduced in infants with respiratory distress, necrotizing enterocolitis, sepsis, or DIC. In the absence of heparin, AT contributes approximately 80% of the thrombin-neutralizing capacity of normal adult plasma. The levels of a second thrombin inhibitor, α 2 -macroglobulin, are higher during the first two decades of life than in adults, and this factor may lessen the risk for thromboembolic complications in AT-deficient patients during childhood.
A number of clinical disorders can be associated with reductions in the plasma concentration of AT, and thus making a definitive diagnosis of the hereditary state is often difficult. Although an AT level in the normal range is usually sufficient to exclude the disorder, low levels should be confirmed at a later date. This determination is ideally performed when the person is no longer receiving warfarin because plasma AT concentrations can occasionally be elevated into the normal range in persons with the deficiency state. In such situations, clinical assessment of the person’s risk for recurrent thrombosis will determine whether discontinuation of warfarin is feasible. In most AT-deficient subjects, however, this effect of oral anticoagulants is not of sufficient magnitude to obscure the diagnosis. Confirmation of the hereditary nature of the disorder often leads to the investigation of other family members. Diagnosis of other affected family members allows appropriate counseling regarding the need for prophylaxis for venous thrombosis and avoidance of prothrombotic risk factors.
Protein C Deficiency
Protein C is a vitamin K–dependent protein synthesized by the liver that circulates as a zymogen. It exerts its anticoagulant function after cleavage to the serine protease activated protein C (APC). This activation process can be mediated by thrombin alone but occurs much more efficiently when thrombin is bound to thrombomodulin on endothelial cells (see Chapter 27 ).
In 1981, Griffin and colleagues described low levels of protein C in a family with recurrent thrombotic events. Subsequently, reports were made of many other families with heterozygous protein C deficiency. Heterozygous protein C deficiency is inherited in an autosomal-dominant fashion; a more severe form of protein C deficiency is an autosomal-recessive disorder. The phenotype of patients with heterozygous protein C deficiency is similar to that of persons with hereditary AT deficiency. In severely affected families, approximately 75% of protein C–deficient persons experienced one or more thrombotic events. The initial episode occurs spontaneously in approximately 70% of persons experiencing such events. The remaining 30% have the usual associated risk factors (e.g., pregnancy, parturition, hormonal contraceptives, surgery, or trauma) at the time that the acute thrombotic events take place. Symptoms occur infrequently until affected persons are in their early twenties, with increasing numbers of persons experiencing thrombotic events as they reach the age of 50 years.
The most common sites of disease are the deep veins of the legs, the iliofemoral veins, and the mesenteric veins. Approximately 63% of affected patients experience recurrent venous thrombosis, and approximately 40% exhibit signs of pulmonary embolism. Superficial thrombophlebitis of the leg veins, as well as cerebral venous thrombosis, can occur in protein C–deficient patients. The literature includes reports of nonhemorrhagic arterial stroke in children and young adults with hereditary protein C deficiency, but a causal relationship is unproven.
The prevalence of protein C deficiency in outpatients who have experienced an initial episode of venous thromboembolism (VTE) ranges from 0.5% to 4%. In earlier reports of more select patient populations, protein C deficiency was more frequently identified and occurred in 2% to 9% of patients. Initial estimates placed the prevalence of protein C deficiency between 1 in 16,000 and 1 in 32,000 within the general population based on the assumptions that protein C was an autosomal-dominant disorder with high penetrance and that at least half the persons with the deficiency would demonstrate symptomatic thrombosis. However, it was difficult to reconcile this figure with the infrequent history of thrombosis in the parents of infants with purpura fulminans, which is due to the homozygous or doubly heterozygous form of protein C deficiency. This disparity led to studies of healthy blood donors in which a much higher prevalence of heterozygosity for protein C deficiency was found, ranging from 1 in 200 to 1 in 500.
The risk of thrombosis initially attributed to protein C deficiency was subject to selection bias in that it was overestimated from familial reports. Data from the Leiden Thrombophilia Study indicate that heterozygous protein C deficiency is associated with about a sevenfold increased risk for an initial episode of deep venous thrombosis compared with persons who have normal protein C activity. Among Italian patients, protein C deficiency is associated with a similar sevenfold increase in venous thrombotic risk. In asymptomatic carriers of protein C deficiency, the incidence of thrombosis is fairly low, at 0.4% to 1.0% annually. Similar to other inherited thrombophilias, this variability in phenotypic expression of protein C deficiency is not explained by differences in the particular genetic defect alone and probably represents a complex interaction with other modulating factors. In pediatric patients, the odds ratio of thrombosis with protein C deficiency is estimated at 7.72 (95% CI, 4.44 to 13.4).
Two major subtypes of heterozygous protein C deficiency have been identified with the use of immunologic and functional assays ( Table 33-2 ). The type I deficiency state is the most common form and is characterized by a similar degree of reduction in both the immunologic and biologic activity of protein C. More than 195 different mutations have been identified, most commonly missense or nonsense mutations. The functional or type II deficiency state is characterized by normal synthesis of an abnormal protein. The functional capacity of protein C is most often assessed by using a snake venom protease (Protac)-based assay to directly activate the protein. After activation, an amidolytic assay can assess the functionality of the catalytic site of the protein. A few persons with normal levels of protein C antigen and amidolytic activity but with substantial reductions in protein C anticoagulant activity have been described. In such cases, protein C anticoagulant activity can be measured with a clotting assay (based on prolongation of the activated partial thromboplastin time [APTT]), and abnormalities presumably reflect a reduced ability of activated protein C to interact with the platelet membrane or its substrates such as factor Va and factor VIIIa. The coagulant assay thus has the highest sensitivity in screening patients for hereditary protein C deficiency.
Activity | |||
---|---|---|---|
Type | Antigen | Amidolytic | Coagulant |
I | Low | Low | Low |
II | Normal | Low | Low |
Normal | Normal | Low |
Levels of protein C antigen in the heterozygous deficiency state overlap with those in the normal population, thus making it difficult to define the diagnosis in some patients. In general, antigen levels of 60% to 70% of normal represent borderline values and warrant repeat testing. Protein C antigen levels can also vary with age. Protein C levels in newborns are 20% to 40% that of normal adult levels, and preterm infants have even lower levels. Neonates with significant perinatal thrombosis can have levels suggestive of homozygous deficiency (<10%). Protein C levels often reach adult levels by 1 year of age (see Chapter 5 ), although further increases are reported into adolescence. In adults, protein C levels typically increase 4% per decade.
Acquired protein C deficiency is found in numerous disease states, including liver disease, DIC, sepsis, and acute thrombosis. A particularly severe form of acquired protein C deficiency has been reported in association with purpura fulminans and DIC in persons with acute meningococcal infections. In contrast to AT, antigenic concentrations of vitamin K–dependent plasma proteins, including protein C, are often elevated in persons with nephrotic syndrome. Most persons with uremia have low levels of protein C anticoagulant activity but normal levels of protein C amidolytic activity and antigen. This situation is attributable to a dialyzable moiety in uremic plasma that interferes with most clotting assays for protein C activity.
As for other γ-carboxylase coagulation proteins (see Chapter 27 ), warfarin therapy reduces functional and, to a lesser extent, immunologic measurements of protein C, thus complicating diagnosis of the deficiency state. Several researchers have proposed that the diagnosis can still be made in this setting on the basis of the ratios of protein C antigen to factor II or factor X antigen. However, this method will not detect patients with type II deficiency, and it can be used only in subjects in a stable phase of oral anticoagulation. Other groups have used protein C activity assays in conjunction with functional measurements of factor VII, a vitamin K–dependent zymogen with a similar plasma half-life. In practice it is preferable to investigate persons suspected of having the deficiency state either before the initiation of oral vitamin K antagonists or after these medications have been discontinued for at least 1 week, and family studies should also be performed. In patients in whom discontinuation of warfarin is impossible because of the severity of the thrombotic diathesis, studies can be performed while they are undergoing therapy with heparin, which does not alter plasma protein C levels.
Warfarin-induced skin necrosis has been associated with the presence of heterozygous protein C deficiency. This syndrome typically manifests during the first several days of warfarin therapy, often in association with the administration of large loading doses of the medication. Skin lesions occur on the extremities, breasts, trunk, and penis and marginate over a period of hours from an initial central erythematous macule. If a product that contains protein C is not administered rapidly, the affected cutaneous areas become edematous, central purpuric zones develop, and the lesions ultimately become necrotic. Biopsies demonstrate fibrin thrombi within cutaneous vessels with interstitial hemorrhage. The dermal manifestations of warfarin-induced skin necrosis are clinically and pathologically similar to those seen in infants who have purpura fulminans resulting from severe protein C deficiency (discussed later).
The pathogenesis of warfarin-induced skin necrosis is attributable to the emergence of a transient hypercoagulable state. Initiation of the drug at standard doses leads to a decrease in protein C anticoagulant activity to approximately 50% of normal within 1 day. Although factor VII activity follows a pattern similar to that of protein C, levels of the other vitamin K–dependent factors decline at slower rates, consistent with their longer half-lives. Increased thrombin generation has been documented in patients during this early phase of warfarin therapy. During this period the drug’s suppressive effect on protein C has a greater influence on the hemostatic mechanism than does its reduction of factor VII. Only about a third of patients with warfarin-induced skin necrosis have an inherited deficiency of protein C, and this complication is a rather infrequently reported event in persons with the heterozygous deficiency state. This syndrome has been reported in association with an acquired functional deficiency of protein C. Reports also have been made of hereditary protein S deficiency and the factor V Leiden mutation in association with warfarin-induced skin necrosis.
The long-established syndrome of purpura fulminans is classically described as a complication of meningococcal infection in children. However, newborns rarely exhibit a syndrome of purpura fulminans, which is associated with laboratory evidence of DIC and protein C antigen levels that are less than 1% of normal. In some instances a history of consanguinity exists in the family, leading to homozygous deficiency of affected infants. Affected newborns can also be double heterozygotes, as was demonstrated in a Chinese patient who had a five-nucleotide deletion in one protein C allele and a missense mutation in the other. The heterozygous parents of infants with purpura fulminans in association with severe protein C deficiency only infrequently have thromboses, in contrast to patients with thrombotic histories and a hereditary partial deficiency of protein C. However, numerous reports have been made of older patients with homozygous or doubly heterozygous protein C deficiency in whom purpura fulminans was not present. These persons generally had protein C levels that were less than 20% of normal in the absence of oral anticoagulant therapy, and their clinical manifestations were similar to those of severely affected subjects from thrombophilic kindreds with the heterozygous deficiency state.
Protein S Deficiency
Protein S is a vitamin K–dependent protein that enhances the anticoagulant effect of APC. Primarily synthesized by hepatocytes but also by endothelial cells, megakaryocytes, and brain cells, protein S serves as a cofactor for APC, which then inactivates factors Va and VIIIa. Factor Va inactivation occurs by an ordered series of peptide bond scissions in the molecule’s heavy chain: first, rapid cleavage at Arg506 takes place, followed by slower cleavage at Arg306 and then Arg679 (see Chapter 27 ). Interaction of protein S with APC results in both an increased affinity for negatively charged phospholipids and a twentyfold enhancement of the slower phase of factor Va inactivation. In plasma, only 40% of protein S is available in the “free” form, whereas the remainder is bound to C4b-binding protein and cannot interact with APC.
Protein S deficiency is an autosomal-dominant disorder that was originally described in 1984 in several kindreds with low levels of protein S and a striking history of recurrent thrombosis. A frequency of thrombosis of approximately 10% has been reported in families with inherited thrombophilia ; however, the prevalence is lower (between 1% and 7%) in consecutive outpatients with a first episode of deep venous thrombosis. Protein S deficiency is generally considered to confer a risk of thrombosis similar to that in persons with protein C deficiency, although the association has been complicated by considerable phenotypic variability.
The clinical findings in patients with heterozygous protein S deficiency are similar to those outlined for deficiencies of AT and protein C. Among 71 protein S–deficient members from 12 Dutch pedigrees, 74%, 72%, and 38% of the individuals sustained deep venous thrombosis, superficial thrombophlebitis, and pulmonary emboli, respectively. The mean age at the first thrombotic event was 28 years, with a range of 15 to 68; 56% of the episodes were apparently spontaneous, and the remainder were precipitated by an identifiable factor. Thrombosis has also been reported in the axillary, mesenteric, and cerebral veins. In pediatric patients with VTE, the OR for thrombosis attributed to protein S deficiency is estimated to be 5.77 (95% CI, 3.03 to 11.0). Although the literature includes case reports of young patients with arterial thrombosis (including arterial ischemic stroke) who were found to have protein S deficiency, current data have not established causation between hereditary protein S deficiency and an increased risk for arterial thrombosis.
Three types of protein S deficiency states can be identified on the basis of measurements of total and free antigen, as well as functional activity ( Table 33-3 ). The type I deficiency state is associated with approximately 50% of the normal total protein S antigen level and greater reductions in free protein S antigen and protein S functional activity. This defect is most often a result of missense mutations and base pair insertions or deletions. The type II qualitative deficiency state, characterized by normal total and free protein S levels but abnormal functional activity, has been identified infrequently, thus suggesting that current functional assays may not screen for all such defects. In persons with type III deficiency, total protein S antigen levels are normal, with disproportionately decreased free protein S and functional activity. Thus initial evaluation for protein S deficiency should test for protein S functional activity.
Type | Protein S Antigen | PROTEIN S ACTIVITY | |
---|---|---|---|
Total | Free | ||
I | Low | Low | Low |
II | Normal | Normal | Low |
III | Normal | Low | Low |
The biologic basis of the type III protein S deficiency state is uncertain. Furthermore, coexistence of type I and type III deficiency has been reported in some protein S–deficient families, which has led to the proposal that the two types of protein S deficiency are phenotypic variants of the same genotype and are not the product of distinct genetic mutations. In a follow-up analysis of one large family that had a high prevalence of both type I and type III deficiency, total protein S antigen levels—but not free protein S antigen levels—were shown to directly correlate with age. These findings were independent of gender and were also seen in nondeficient family members. The researchers concluded that a single point mutation in the protein S gene was responsible for the quantitative type I deficiency but that the type III phenotype was actually due to an age-dependent free protein S deficiency; with increasing age, the relative concentrations of free protein S to total protein S decreased. However, in a Dutch cohort study of first-degree family members with protein S deficiency it was found that affected relatives of probands with type I deficiency were at increased risk for VTE, whereas affected relatives of probands with type III deficiency were not at increased risk for VTE.
Acquired protein S deficiency has multiple causes, including pregnancy and oral contraceptive use. Protein S levels are commonly low in inflammatory states, including DIC and acute thrombosis, largely because of protein S interaction with C4b-binding protein. C4b-binding protein is an acute phase reactant and shifts protein S to the complexed, inactive form, which leads to decreased protein S activity. Levels of total and free protein S are significantly reduced in men infected with human immunodeficiency virus. Although total protein S antigen measurements are generally increased in persons with nephrotic syndrome, functional assays are often reduced because of loss of free protein S in urine and elevated C4b-binding protein levels.
The lower limit of normal total and free protein S in plasma is approximately 65%; however, considerable overlap exists between the heterozygous deficiency state and levels at the low end of the normal range. Such overlap is largely due to the influence of age, which leads to an increase in protein S levels, and the influence of gender, with females having a lower normal limit for plasma protein S levels compared with males. Thus it is difficult to diagnose heterozygous protein S deficiency by performing a single assay; repeat sampling and family studies are usually required to make the diagnosis.
An epidemiologic study demonstrated that adults with low protein S levels and a negative family history of venous thrombosis have minimal, if any, increase in risk of a first episode of VTE. Among 5317 patients and control subjects evaluated, a free protein S level below the 2.5th percentile (i.e., <53 units/dL) did not confer an increased risk of thrombosis compared with a higher free protein S level (OR, 0.82; 95% CI, 0.56 to 1.21). A free protein S level below the 0.10th percentile (i.e., <33 units/dL) was associated with an increase in risk of VTE that was not statistically significant (OR, 5.4; 95% CI, 0.61 to 48.8). Total protein S levels did not correlate with thrombosis, even below the 0.10th percentile. The results of this study imply that the increased risk of VTE is minimal in persons with protein S deficiency in the absence of a family history.
Young patients with recurrent VTE disease associated with doubly heterozygous or homozygous protein S deficiency have been identified. The parents of these patients were asymptomatic and had laboratory studies consistent with type I protein S deficiency. Neonatal purpura fulminans has been described in association with homozygous protein S deficiency.
Resistance to Activated Protein C and the Factor V Leiden Mutation
Before 1993, hereditary deficiencies were infrequently identified in patients with familial thromboembolic disease. Dahlbäck and colleagues identified several probands with venous thrombosis that appeared to be resistant to APC in an APTT-based clotting assay. When compared with control subjects, many family members of the probands also had a blunted anticoagulant response to APC. The following year, Bertina and colleagues identified the genotype underlying most cases of APC resistance as a single point mutation in the factor V gene, leading to substitution of Arg506 by glutamine. This mutation at an APC cleavage site renders factor Va relatively resistant to inactivation by APC.
After the description of APC resistance by Dahlbäck and colleagues, several studies revealed that this defect was relatively common in persons with venous thrombosis. In 1994 Svensson and Dahlbäck screened 104 consecutive Swedish persons referred for evaluation of venous thrombosis and found that 33% of the subjects demonstrated APC resistance. In a U.S. referral population of persons younger than 50 years who had unexplained VTE disease, Griffin and colleagues found that approximately 50% exhibited APC resistance.
Dutch investigators had previously initiated the Leiden Thrombophilia Study to identify risk factors for a first episode of venous thrombosis. Excluding patients with known malignancies, 345 consecutive outpatients younger than 70 years who had confirmed deep venous thrombosis were initially reported. With use of a screening APTT-based assay, APC resistance was identified in 21% of persons with thrombosis and in 5% of age- and sex-matched healthy control subjects. The factor V Arg506Gln (or factor V Leiden) mutation was found in more than 80% of the patients who had been found to be APC resistant. The lower frequency of APC resistance identified in the Leiden Thrombophilia Study compared with previous studies is attributable to differences in selection criteria and referral cohorts.
The U.S. Physicians’ Health Study has also provided valuable data regarding factor V Leiden as a risk factor for venous and arterial thrombosis. In a retrospective case-control study of 14,916 healthy men older than 40 years with a mean follow-up period of 8.6 years, heterozygosity for the factor V Leiden mutation was identified in 12% of patients (14/121) who had a first episode of deep venous thrombosis or pulmonary embolism and in 6% of control subjects. The relative risk for VTE was increased 3.5-fold in persons with no other concomitant risk factors but was reduced to 1.7-fold in persons who had preexistent cancer or had recently undergone surgery. This study also showed that elderly patients with venous thrombosis frequently have the mutation. Among men older than 60 years who had initial episodes of venous thrombosis and no identifiable triggering factors, 26% (8/31) were heterozygotes for factor V Leiden mutation.
A cohort study of more than 9000 randomly selected adults in Denmark found that the simultaneous presence of smoking, obesity (body mass index >30 kg/m 2 ), older age (>60 years), and the factor V Leiden mutation resulted in absolute 10-year VTE rates of 10% in heterozygotes and 51% in homozygotes.
A systematic review and meta-analysis of pediatric studies found that factor V Leiden heterozygosity conferred an odds ratio of 3.77 (95% CI, 2.98 to 4.77) for a first episode of VTE and 0.64 (95% CI, 0.35 to 1.18) for recurrent VTE in children. In a cohort of children who had spontaneous VTE, factor V Leiden still did not increase the risk of recurrent thrombosis (OR, 1.35; 95% CI, 0.91 to 1.98). A systematic review and meta-analysis of observational studies, including pediatric patients with cerebral sinovenous thrombosis, reported an OR for thrombosis of 2.74 (95% CI, 1.73 to 4.34) in factor V Leiden mutation carriers.
A prospective cohort study determined the incidence of VTE in asymptomatic carriers of the factor V Leiden mutation who were identified through family studies of symptomatic probands. Nine events occurred in 1564 observation-years, for an annual incidence of 0.58%. It was concluded that the absolute annual incidence of VTE in asymptomatic carriers of the mutation is low.
A number of studies have examined whether factor V Leiden mutation, a prevalent abnormality in white populations, leads to an increased risk for arterial thrombotic events. No convincing data are available to indicate that other thrombophilic states such as deficiencies of AT, protein C, and protein S confer an increased risk for arterial thrombosis, but evaluation of these associations is complicated by the relative infrequency of these defects. In a cohort of men older than 40 years who had a low prevalence of smoking in the U.S. Physicians’ Health Study, an association was not found between the factor V Leiden mutation and myocardial infarction or stroke. In a younger cohort of Italian patients who experienced myocardial infarction before 45 years of age, an increased incidence of the factor V Leiden mutation relative to that in control subjects also was not found. Like other hereditary thrombophilic states, factor V Leiden has been associated with pediatric arterial ischemic stroke, although data are lacking to prove causation.
Because of the frequency of heterozygosity for the factor V Leiden mutation in white persons, homozygosity is not a particularly rare occurrence, and its presence is compatible with normal gestational survival. However, homozygotes for factor V Leiden are at significantly higher risk for venous thrombosis than are heterozygotes and demonstrate heightened APC resistance in APTT assays. Patients with heterozygosity for the factor V Leiden mutation along with deficiencies of protein C, AT, or protein S are also at significantly greater risk for VTE than are persons who have only a single genetic defect. The genes for factor V and AT are both located on the long arm of chromosome 1, thereby allowing coinheritance of the factor V Leiden mutation and an AT mutation within all affected members of a family. This situation is expected to lead to an even more severe thrombotic diathesis.
Obstetric complications such as severe preeclampsia, abruptio placentae, fetal growth retardation, and stillbirth are associated with intervillous or spiral artery thrombosis and consequent placental insufficiency. Factor V Leiden, as well as other hereditary thrombophilias, is associated with an approximate tripling of the risk for late fetal loss. In contrast, first-trimester loss does not correlate with factor V Leiden or other hereditary thrombophilias. An increased incidence of factor V Leiden, as well as other thrombophilias, was also reported in women in association with other obstetric complications ; however, associations with preeclampsia and intrauterine growth restriction/retardation have not been corroborated.
The prevalence of heterozygosity for the factor V Leiden mutation in white persons, including European, Jewish, Israeli Arab, and Indian populations, ranges between 1% and 8.5%. The mutation apparently is rare (<1%) in African blacks and in Chinese, Japanese, or Native American populations. In Europe, the mutation has been found to be more prevalent in northern countries such as Sweden than in southern countries such as Spain and Italy. By using dimorphic sites in the factor V gene to perform haplotype analysis, data have been provided that support the existence of a single founder allele among whites of differing ethnic backgrounds. It was also estimated that the mutation originated approximately 30,000 years ago, after the evolutionary divergence of white, African, and Asian populations.
Two mutations at the Arg306 residue in factor V, the second APC cleavage site in the activated cofactor, have been described in patients who have a history of thrombosis. These mutations are replacement of Arg306 with threonine (factor V Cambridge) and with glycine (in Chinese persons from Hong Kong). The latter mutation, however, evidently has no clinical relevance because it is not associated with APC resistance and the mutation is as common in healthy Chinese blood donors as it is in patients with thrombosis (4.5% and 4.7%, respectively). The factor V Cambridge mutation is extremely rare among white persons with VTE disease.
Co-segregation of heterozygous APC resistance as a result of the factor V Leiden mutation and type I factor V deficiency has been reported in some patients. The plasma of these persons manifests severe APC resistance in partial thromboplastin time (PTT) assays, as found in homozygous factor V Leiden patients (i.e., the patients with such co-segregation are pseudohomozygous). These patients were seemingly more prone to thrombosis than were heterozygous relatives with factor V Leiden alone, thus suggesting that their clinical phenotype is similar to that of homozygous factor V Leiden patients.
Several polymorphisms are present in the factor V gene. An extended factor V gene haplotype (HR2) containing the R2 polymorphism (His1299Arg) is associated with mild APC resistance and occurs with increased frequency in heterozygous patients with the factor V Leiden mutation and the lowest APC resistance ratios. Although one case-control study found that the R2 allele was a risk factor for VTE with an OR of 2.0 after excluding subjects with genetic defects such as factor V Leiden, another case-control study found no significant increase in risk.
The initial observations of Dahlbäck and colleagues facilitated the development of a PTT-based assay that serves as a screening test for APC resistance. The PTT assay is performed in the presence or absence of a standardized amount of APC, and the two clotting times are converted to an APC ratio. Results can be interpreted by comparing the ratio with the normal range or by normalizing it to the APC resistance ratio obtained with normal pooled plasma. Although this first-generation APC resistance assay was conceptually quite simple and easy to perform in a coagulation laboratory, it required careful standardization and determination of the normal range in at least 50 control subjects. The level of APC, the PTT reagent, and the instrumentation used for clot detection affected the performance characteristics of the assay. Some assays using this format therefore had inadequate sensitivity and specificity for the factor V Leiden mutation. Moreover, patients who were receiving anticoagulants or had an abnormal PTT because of other coagulation defects could not be evaluated with this assay, and the test was not validated in patients with acute thrombosis or in pregnant women.
The discovery that factor V Leiden is the dominant genetic defect responsible for APC resistance facilitated the development of second-generation coagulation tests that, with proper standardization, can provide nearly 100% sensitivity and 100% specificity for detection of the mutation. For these tests, patient plasma is diluted in a sufficient volume of factor V–deficient plasma, and a PTT-based assay is then performed. This modification also permits evaluation of the plasma of patients who are receiving anticoagulants or have abnormal PTT results because of coagulation factor deficiencies other than factor V.
Because the dominant mutation underlying APC resistance is factor V Leiden, diagnosing this defect by analyzing genomic deoxyribonucleic acid (DNA) in peripheral blood mononuclear cells is an attractive option. This analysis can be readily accomplished by amplifying a DNA fragment containing the factor V mutation site with polymerase chain reaction.
Prothrombin G20210A Mutation
In 1996, investigators from Leiden reported that a G-to-A substitution at nucleotide 20210 in the 3′-untranslated region of the prothrombin gene is associated with a 30% elevation of plasma prothrombin levels and an increased risk for venous thrombosis. This mutation, which was discovered by directly sequencing the prothrombin gene of selected patients with venous thrombosis, is located in the 3′-untranslated region at the cleavage site for polyadenylation of prothrombin mRNA. The prothrombin G20210A mutation changes the position of the 3′-cleavage/polyadenylation reaction in prothrombin mRNA, thereby leading to increased prothrombin biosynthesis by the liver ; this finding contrasts with previous data indicating that the mutation changes mRNA stability by increasing mRNA 3′-end formation.
Investigation of a referral population with a personal and family history of venous thrombosis demonstrated that 18% had the mutation in the 3′-untranslated region of the prothrombin gene, whereas it was present in only 1 of 100 healthy control subjects. Among these patients with thrombosis, 40% also carried the factor V Leiden mutation, thus emphasizing the current view of venous thrombosis as a multigene disorder.
In the Leiden Thrombophilia Study, 6.2% of patients with venous thrombosis and 2.3% of healthy matched control subjects had the prothrombin gene mutation. This mutation independently confers a 2.8-fold increased risk for venous thrombosis, and the effect is operative in both sexes and all age groups. Among heterozygotes with the prothrombin gene mutation, 87% of patients with thrombosis and control subjects in the study had prothrombin activity levels that were greater than 1.15 units/mL, whereas only 23% of those with a normal prothrombin genotype had levels elevated to this degree. A systematic review and meta-analysis of pediatric studies found that prothrombin gene mutation G20210A heterozygosity conferred an OR of 2.64 (95% CI, 1.60 to 4.41) for a first episode of venous thromboembolism and an OR of 1.88 (95% CI, 1.01 to 3.49) for recurrent VTE. A similar analysis of cases of cerebral sinovenous thrombosis in children found that prothrombin mutation carriers had an OR for thrombosis of 1.95 (95% CI, 0.93 to 4.07). An association with arterial ischemic stroke was also observed.
In numerous other studies the prothrombin G20210A mutation has been documented to have a significantly higher frequency in patients with venous thrombosis than in healthy control subjects. Large population studies have shown an overall prevalence of about 2% in the general white population, with significant geographic variation. In southern Europeans the prevalence is about 3%, but it was only very rarely identified in persons of Asian or African descent.
Polymerase chain reaction methods are used to detect the prothrombin G20210A mutation in genomic DNA. Although plasma prothrombin activity and antigen levels are significantly higher in persons with the prothrombin G20210A mutation, prothrombin concentrations cannot be used to screen for the defect because of significant overlap with the normal population.
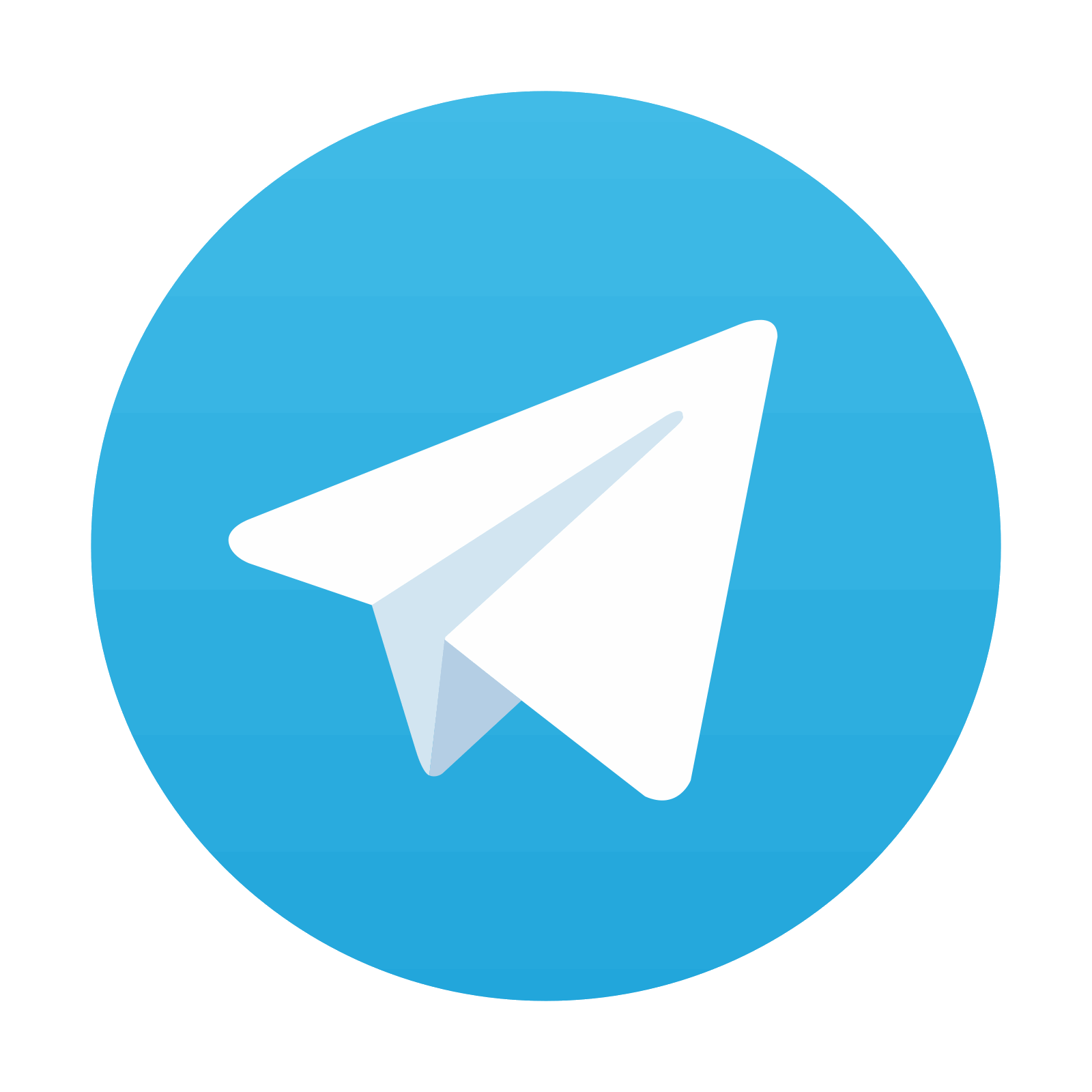
Stay updated, free articles. Join our Telegram channel
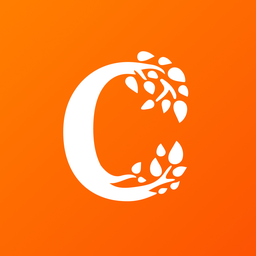
Full access? Get Clinical Tree
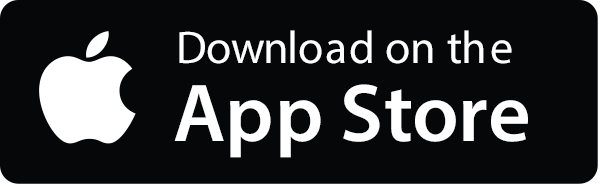
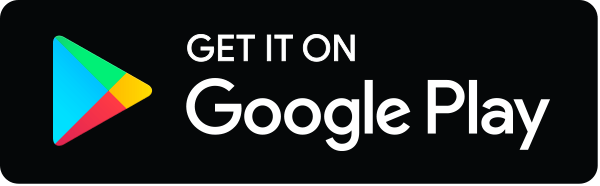