Model
Tumor stage
Initiation
Promotion
Progression/metastasis
Adenocarcinoma
Pro-inflammatory mediators
Transcription factors
NFκB, NRF2
NFκB
NFκΒ, EGR-1
Cytokines/chemokines
TNF, IL4Rα, IL17
IL6, IL17
MCP-1, MIF
Eicosanoid pathway
cPLA2
EP2 (PGE2)
Nuclear receptor
PPARγ
Signal transduction
K-Ras
Other
iNOS, MMPs, KEAP-1
Anti-inflammatory mediators
Transcription factors
NRF2, MIG, IP10
Cytokines/chemokines
IL10
IFNγ
Eicosanoid pathway
PGI2
PGI2
Nuclear receptor
PPARγ
Innate immune receptor
TLR4
Mucinous ADC
Pro-inflammatory
Transcription factor
NKX2-1/KrasG12D
Squamous cell carcinoma
Pro-inflammatory
NFκB pathway
IKKKa/Ka
In contrast, in prostaglandin E2 receptor EP2 subtype (EP2)-deficient mice, one of the receptors for PGE2, developed significantly less tumors than respective wt controls [98]. Interestingly, microsomal PGE synthase mPGES-1 lung-specific (SPC-promoter driven) overexpressing mice that produce elevated levels of PGE2, were not more susceptible to pulmonary carcinogenesis [99]. The finding from these two studies is perplexing, however, multiple isoforms of PGES exist, several PGE2 receptors, as well as cell specificity of the models (epithelial versus whole body), may explain some of these discrepancies. A delicate balance between PGI2:PGE2 levels likely determines the inflammatory nature of this pathway. When both COX-1 and COX-2 are inhibited (either pharmacologically or genetically), several prostaglandins are inhibited (i.e., (PGI2 and PGE2) which may potentially nullify or decrease the effectiveness of the drug. However, as will be discussed below, it may depend on timing of treatment with inhibitors of these pathways.
Peroxisome Proliferator-Activated Receptor-γ
Peroxisome proliferator-activated receptor-γ, a nuclear receptor subfamily member, is a ligand-activated transcription factor with two human isoforms (γ1 and γ2). It is involved in many physiological processes, such as adipocyte development (induces adiponectin), and is expressed in other tissues as well as cancer cell lines, such as lung [100–102], and inhibits TNF, NO, IL6, as well as proliferation of tumor cells [103]. Some PPARγ SNPs in lung are associated with decreased lung cancer risk, while others are associated with increased risk [104]. PPARγ is expressed in multiple lung cell types, including epithelial cells, immune cells, such as macrophages, endothelial cells, and fibroblasts.
In NSCLC cell lines, overexpression of PPARγ inhibited anchorage-independent growth and promoted differentiation [105]. In lung-specific PPARγ-overexpressing mice, pulmonary tumorigenesis, as well as suppression of COX-2 via NFκB, was significantly inhibited (75 % reduction in tumor multiplicity) [106]. However, if bone marrow from mice lacking PPARγ specific to myeloid cells (Lys-M-Cre+/PPARγflox/flox mice) was transplanted into wild-type mice, there was significant decrease in secondary tumors that were not altered by pioglitazone, a PPARγ agonist [107]. In addition, arginase-positive (a M2 macrophage marker) cells were reduced in the lungs supporting decreased M2 macrophages. Fewer metastases were also observed in an orthotopic mouse model after PPARγ deletion in the myeloid cells using LOX recombination [107]. These M2 alternative macrophages are hypothesized to promote tumor progression through induction of angiogenesis , breaking down the matrix, and increasing tumor cell motility, likely linked to the other two factors [107]. These findings suggest that PPARγ plays dual roles in lung tumorigenesis, both pro- and antitumorigenic, depending on tumor stage studied: antitumorigenic during early stages (initiation/promotion) and pro-tumorigenic during tumor progression and metastasis. We refer readers to go through a more complete review of PPARγ in lung cancer development.
Adiponectin (Acrp30) is an adipocyte-derived protein that is anti-inflammatory and important in regulating fat and glucose metabolism [108]. PPARγ is a central regulator of adiponectin [102]. In COPD, plasma levels of adiponectin are considered a biomarker for certain COPD phenotypes [109]. Adiponectin is also elevated in the BALF from COPD patients [110] and is protective against tobacco-induced inflammation and emphysema in Acrp30-deficient mice [111]. In Acrp30-deficient mice compared to wild-type mice injected with the Lewis Carcinoma cells, the deficient mice were found to promote tumor growth linked to reduction of macrophage recruitment to the site of the tumors [112]. Adiponectin is increased in some COPD patients that are underweight and does appear to be related inversely with body mass index (BMI) [65, 113]. Thus, the protective role of adiponectin in lung cancer may be a potential future therapeutic target.
Nuclear Factor Kappa B (NFκB)
The NFκB transcription factor is involved in many signaling pathways, including those for proliferation, apoptosis, and differentiation [114]. In vivo models, such as hepatocarcinogenesis and colitis-associated cancer [115–117], demonstrate how NFκB can act as a tumor promoter. In squamous cell carcinomas, however, NFκB appears to protect against neoplastic development [118–120]. IKKα, IKKβ, and IKKγ are required for NFκB activation, where the IKK complex phosphorylates ΙκΒα targeting it for subsequent ubiquitization, and releasing NFκB to translocate from the cytoplasm to the nucleus and affect gene transcription [114]. In a hepatocyte-specific IKKβ-deficient mouse model, the IKKβ−/− mice developed a marked increase in tumor development compared to wt controls in chemically induced hepatocarcinogenesis [116, 121]. However, hepatocytes and Kupffer cells-specific IKKβ-deficient mice had significantly reduced chemically induced hepatocarcinogenesis [116]. Thus, carcinogen, tissue and cell specificity of the models can alter the outcome of responsiveness in mice deficient in the NFκB pathway mediators.
Mouse strains that are susceptible to lung carcinogenesis, such as FVB or BALB/c, have significantly elevated levels of NFkB in response to urethane in the airway epithelium, type II cells, and macrophages compared to a resistant strain, B6 [122]. The same study also used mice overexpressing, by a Clara cell-specific promoter (CC10), IKBα, an inhibitor of NFκB, in the airway epithelial cells using a Clara cell-specific promoter (CC10) to demonstrate that the inhibition of NFκB greatly decreased lung tumor multiplicity in urethane-induced carcinogenesis compared to those observed in wt mice. In this study, the authors also demonstrated that inflammatory cells (macrophages, PMNs) and cytokines (TNF and IL12p70) were inhibited in the lungs of mice overexpressing IKBα. It is therefore expected that other pro-inflammatory mediators downstream of NFκB are involved in this mechanism (see below). In a second model, transgenic mice overexpressing constitutive IκB kinase β (IKKβ) in the airway epithelium (IKTA mice) were used to provide continual activation of NFκB in the airways of both FVB and B6 backgrounds [123]. These mice exhibited chronic inflammation, PMNS, macrophages, and lymphocytes, without stimulus. Following a single urethane injection, the IKTA mice developed more tumors and inflammation (macrophages, lymphocytes) compared to WT mice, but no differences in tumor size or BrDu uptake, reflecting proliferative rates, were observed [123]. However, increased numbers of early lesions, atypical adenomatous hyperplasias [124], were observed, supported by increased PCNA staining and decreased caspase 3, in the IKTA mice compared to WT mice. T regulatory (Treg) cells were upregulated in the IKTA mice along with the NFKB-induced chemokines that regulate Treg recruitment (CCL17, 20, and 22) [123]. In addition, when the MCA/BHT two-stage model was used with these mice on an FVB background, IKTA mice were found to significantly promote tumor development above that observed with MCA/BHT alone in WT mice. These IKTA mice were also able to promote tumors in the absence of BHT, suggesting that NFκB can act as a tumor promoter [123]. Thus, active NFκB signaling in the lung can alter the lung to create a pro-tumorigenic microenvironment. Interestingly, bortezomib, a proteosome inhibitor used to inhibit NFκB activation, increased tumor number and size in response to urethane while inhibiting NFκB expression in respiratory epithelium and macrophages, 4 months following urethane [66]. These results were surprising, however, likely due to a continuation of inflammation, characterized by elevated macrophages, PMNs, and lymphocytes, as well as multiple cytokines/chemokines, in chronic bortezomib-treated animals. Bortezomib was evaluated in clinical trials for NSCLC and was found to have little effect [125, 126]. An important point here is that this drug is not specific to NFκB and has alternative target pathways.
Additional studies examined the role of NKFB in metastasis. LLC cells metastasized more extensively in the lungs of mice administered adenoviral vectors encoding active NFκB, with increased NFκB activity and hence enhanced lung inflammation prior to tail vein injection of the LCC cells [127].
NRF2/KEAP1 Pathway
Nuclear factor (erythroid derived 2)-like 2 (NRF2) is a master transcription factor that regulates antioxidant response element (ARE)-mediated expression of antioxidant enzymes and cytoprotective proteins [128]. Excess reactive oxygen species (ROS) cause oxidative damage to cellular DNA, lipids, and proteins; genetic changes and/or epigenetic alterations can lead to the dysregulation of oncogenes and tumor suppressor genes, ultimately contributing to the pathogenesis of cancer [129, 130]. To alleviate this oxidative stress, there are several antioxidant stress responses, many regulated by NRF2. NRF2 expression is abundant in tissues including the lung [131] where detoxification reactions occur and under normal physiological conditions it interacts with its own negative regulator, Kelch-like ECH-associated protein 1 (KEAP1) [132]. KEAP1 is a cytoplasmic, cysteine-rich, actin-bound protein that sequesters NRF2 in the cytoplasm for eventual ubiquitinylation and degradation [131–133]. During oxidative stress, KEAP1 cysteines are oxidized, leading to a disruption of the KEAP1-NRF2 complex and the release of NRF2. NRF2 translocates to the nucleus to transcribe genes encoding various antioxidant proteins and phase II detoxifying enzymes [133]. Alternative pathways for NRF2 activation are through the phosphorylation of NRF2 by protein kinase C (PKC) or RNA-dependent protein kinase R (PKR)-like endoplasmic reticulum kinase (PERK), resulting in the release of NRF2 from KEAP1 [134–136].
The roles of NRF2 and KEAP1 in lung cancer are controversial [132, 137–140]. Lung cancer differs from findings in most other organs and pulmonary diseases, such as respiratory syncytial virus infection [141], emphysema [142], and hyperoxia [143]. In the aforementioned respiratory diseases, symptoms significantly worsen in the absence of NRF2. Interestingly, the tumor responses appear unique in mechanism compared to other diseases. Other mouse models (including colon, bladder, liver, and mammary) have demonstrated that a lack of NRF2 increases the potential for carcinogenesis [144–147].
Animal studies on the NRF2 pathway have used NRF2-deficient mice on multiple backgrounds, Keap1-deficient mice, metastasis models, as well as NRF2-deficient mice crossed to a K-Ras G12D mouse. These models will be discussed next. Urethane-induced tumorigenesis resulted in significantly less tumor development in NRF2-deficient mice (−/−) than the NRF2 +/+ mice (WT; BALB background strain) [148]. However, NRF2 −/− mice had enhanced inflammatory cell infiltrates including monocytes, macrophages, and lymphoctyes, hyperpermeability, and elevated myeloperoxidase, that was suggestive of increased numbers of PMNs, compared to the NRF2 +/+ mice 11 weeks following urethane. Additionally, NRF2 +/+ mice had early adenomatous lesions 12 weeks following urethane above that observed in the NRF2 −/− mice, while the NRF2 −/− mice had increased apoptotic cells compared to the wild-type mice [148]. Finally, cell death markers, such as LDH, support the hypothesis that the urethane-initiated epithelial cells, such as the type II cells, were more susceptible to cell death in the mice lacking Nrf2. The NRF2 +/+ mice, therefore, have both a growth advantage and an increased cytoprotection for tumorigenesis.
Transcriptome analysis revealed differences between the NRF2 −/− and NRF2 +/+ mice involved in these responses to urethane at an early and late time point. Early (12 weeks) NRF2-modulated genes involved glutathione metabolism, cell–cell signaling, oxidative stress, and immune responses [148]. At the advanced stage, the NRF2-dependent genes associated with cell cycle/proliferation and cell death, which correlated in direction and magnitude with the increased death of initiated cells in the NRF2 −/− mice. In this primary mouse cancer model, NRF2 promotes survival properties and therefore supports the human studies demonstrating resistance to anticancer drugs as well as increased malignancy. NRF2 protection may be both carcinogen and stage dependent since studies using the two-stage MCA/BHT model with NRF2 −/− mice did not demonstrate differences in tumor numbers between strains (unpublished data, A. K. Bauer and H. Y. Cho).
The concept of ROS reduction leading to increased carcinogenesis is against the normal dogma [149]. However, oncogenes K-Ras G12D or B-Raf V619E (mutated, activated) expressed in vitro in murine NIH3T3 or mouse embryonic fibroblasts (MEFs) resulted in decreased ROS levels [150]. In contrast, Nrf2 activity was elevated while the ratio of reduced to oxidized levels of glutathione (GSH/GSSH) increased [150]. K-Ras and B-Raf signaled through MEK, ERK MAP Kinase, and AP-1, eventually activating Nrf2, leading to antioxidant responses [150]. In vitro studies were validated in several in vivo mouse models (lung and pancreatic cancer). NRF2 −/− and NRF2 +/+ mice were bred to the K-Ras G12D mouse (B6/129/SJL background strain) in lung tumor studies which demonstrated that the NRF2 −/− mouse had a significant reduction in K-Ras G12D-initiated lung tumors compared to WT mice [150]. Ki67 staining was also reduced, indicating less proliferation and overall decrease in adenomas, adenomatous alveolar hyperplasias, and bronchiolar hyperplasias [150]. Thus, these studies suggest that Nrf2 can be regulated by specific oncogenes (Kras, B-raf) to enhance tumorigenesis by reduction of ROS creating a more favorable cellular microenvironment. The findings described here support the other primary mouse study which suggests that initiated cells are reduced in mice lacking NRF2. Less initiated cells lead to a decreased number of tumors. Thus, the cellular environment in the Nrf2−/− mice lacks the favorable protection of mice with sufficient NRF2 [148].
Lastly, NRF2-deficient mice on a C57/BL6/J background developed a significantly higher number of metastatic LLC lung nodules than wild-type mice [151]. Inflammation, both pulmonary and bone marrow (myeloid-derived suppressor cells; MDSCs), was also elevated in the NRF2-deficient mice with metastatic tumors in the lung. KEAP1 mutant mice with increased levels of Nrf2 protein were resistant to metastasis [151] and demonstrated reduced levels of ROS. In metastasis, Nrf2 appears to be preventive, which is the reverse of findings observed in the primary mouse lung cancer studies. However, the mechanisms regulating metastasis differ from the earlier stages of carcinogenesis. Another difference between studies was the strain backgrounds which are known to differ in many phenotypes, including the polarity of their immune systems, which could influence responsiveness [152].
Cytokines
Several cytokine models have been used in primary mouse tumor models. Tumor necrosis factor α (TNF) is a pro-inflammatory cytokine that induces other inflammation pathways [153]. TNF is involved in many components of tumorigenesis, from initiation through malignancy [153]. TNF heterozygous (−/+) mice developed significantly less lung tumors compared to the wt controls following urethane-induced carcinogenesis (Table 4.1). One of the major quantitative trait loci (QTL) sites identified for pulmonary carcinogenesis models is on chromosome 17, as well as most pulmonary inflammation models [25], and TNF resides within this QTL. Interestingly, TNF is clustered with the two lymphotoxin (LT; Ltα and LTβ) genes, which can also bind to TNF receptors, in addition to the LT receptor [154–156], all of which are important in the inflammation models, such as ozone exposure. Thus, the involvement of the TNF cluster in pulmonary carcinogenesis is likely.
Interleukin 6 (IL6) can be elevated in human COPD and is considered a pro-inflammatory cytokine linked to lung cancer [157, 158]. Il6-deficient mice bred to the CCLR mice (KrasG12D) were used alone and in the NThi COPD-like model [69]. These mice with no stimulus developed fewer tumors than the CCLR mice (WT) alone. Additionally, when exposed to NThi, these mice exhibited significant reductions in promotion, suggesting IL6 is a critical cytokine in the inflammation observed in response to NThi, a COPD-like model.
IL10 is an anti-inflammatory cytokine, based on suppression of macrophage and dendritic cell (DC) function, including production of pro-inflammatory cytokines and antigen presentation, Th2 cytokine [159]. However, feedback mechanisms of both the Th1 and Th2 responses can result from suppression. In UV-induced skin carcinogenesis, IL10-deficient mice were found to be resistant [160]. IL10 is immunosuppressive to CD8 + T cell effector functions and DC maturation, which can lead to an impaired antitumor responses [159, 161]. Clinically, recombinant IL10 suppressed endotoxin and resulted in immune stimulatory capacities as well as anti-inflammatory roles [159]. NSCLC patients expressing elevated IL10 levels in tumor-associated macrophages (TAM) were associated with a worse prognosis [162]. In urethane-induced carcinogenesis, Il10 heterozygous (−/+) mice enhanced lung tumor multiplicity in female mice only compared to wt controls [163]. Therefore, IL10 appears to both suppress pro-inflammatory pathways, such as TNF, and impair antitumor responses. Additional IL10 tumor models demonstrated that lower IL10 levels appear immunosuppressive while higher IL10 levels may induce immune activation events, such as tumor rejection [159]. Gender also appears to be involved in lung IL10 responses; the mechanism behind gender involvement is unclear. Thus, the tumor microenvironment in addition to the amount of IL10 may determine the outcome of the response.
IL17 is a CD4 helper T cell-derived pro-inflammatory cytokine that is involved in upregulating cytokines and chemokines [164]. Increases in IL17 have been linked to asthma, COPD, and several types of tumors, including lung ADC. In the CC-LR and CC-LR-NTHi mouse models, IL17 was significantly elevated [165]. IL17-deficient mice crossed to the CC-LR (mutant K-ras) mice in the presence or absence of NTHi developed far fewer tumors than the wild-type mice [165]. Inflammatory responses in these Il17-deficient mice were also greatly reduced [165] as well as the cytokines regulated by IL17, such as Il6, Cxcl2, Ccl2, and Arg1. Additionally, l17-deficient mice had reduced levels of myeloid-derived suppressor cells (MDSCs) or Gr1 +Cd11B + cells, which were mostly Ly6G+, indicating mostly PMN-like MDSCs. Lastly, CC-LR mice administered anti-Gr1 also resulted in reduced numbers of tumors and inflammation, indicating these PMN-like MDSCs are involved in the regulation of pro-tumorigenic inflammation.
Pulmonary Protection Through the Innate Immune system
The toll-like receptors (TLRs) are the most commonly studied receptors in innate immunity. There are now 13 of these receptors, although the function of 10, 12, and 13 is unknown. TLR4 can both exacerbate and inhibit pulmonary inflammation and injury. For example, TLR4 exacerbates ozone-, and lipopolysaccaride (LPS)-induced lung injury and inflammation [166, 167]. TLR4 protects against ovalbumin-induced and oxidant-induced (hyperoxia) pulmonary inflammation and injury [168–171]. Additionally, TLR4 confers protection against gastric and cutaneous carcinomas, in humans and mice, respectively [172, 173]. C3H/HeJ (TLR4 dominant negative mice) cannot present antigen and instead degrade the antigenic material from phagocytosed dying tumor cells [174]. In addition, DCs from humans with a nonfunctional TLR4 mutation exhibited a marked reduction in antigen presentation [174]. Functional TLR4 versus mutated TLR4 (either 299 or 399 mutations) also provided sigificantly longer metastasis-free survival in breast cancer patients [174].
Lung cancer risk is significantly decreased in those individuals exposed to endotoxin, such as farm and textile workers [175–179]. The primary receptor that binds endotoxin (lipopolysaccharide; LPS) is TLR4 [167], thus it is likely involved in the protection observed with endotoxin exposure. BHT-induced inflammation (macrophages and lymphocytes) and injury was significantly higher in Tlr4-deficient mice than the wt controls [180]. Additionally, Tlr4-deficient mice were more susceptible to tumor multiplicity compared to wt mice 20 weeks following the MCA/BHT protocol. Thus, in the MCA/BHT model, TLR4 appears to protect the pulmonary epithelium possibly by reducing the inflammation and pulmonary injury elicited by the oxidative metabolites of BHT. In other types of cancers, other TLR agonists, such as for TLR7 or TLR8 (synthetic imidazoquinoline, imiquimod, resiquimod, etc.), are approved for treatment of basal cell carcinoma and potentially for breast and melanoma [181]. Thus, these innate immune receptors should not be overlooked for future therapeutic strategies in lung.
Interferon γ is a Th1 cytokine and a key immunoregulatory mediator involved in eliciting innate and adaptive responses in host defense [182, 183]. IFNγ can activate macrophages, upregulate MHC class II molecules, and stimulate natural killer cells [184]. Specifically, IFNγ increases nitric oxide synthase [167], indoleamine (2,3)-dioxygenase(tryptophan degradation), and reactive oxygen species (ROS) [184]. MCA-induced fibrosarcoma was significantly enhanced in Ifnγ-deficient mice compared to wt controls [185]. Additionally, some human tumors are unresponsive to IFNγ [185], which supports a more general antitumorigenic role of this cytokine. Ifnγ –deficient mice developed significantly more and larger tumors than wt mice in response to the MCA/BHT model (A. K. Bauer and S. R. Kleeberger, unpublished results). BHT-induced chronic inflammation in the Ifnγ-deficient mice was also significantly elevated compared to wt controls. In a separate study, Ifnγ-deficient mice stimulated tumor growth in urethane-induced carcinogenesis [186]. In human cancers including NSCLC, IFNγ upregulates antiangiogenic chemokines such as monokine-induced by IFNγ (MIG; CXCL9) and interferon-activated p10 (IP10; CXCL10) [187–190]. These studies on specific innate immune pathways imply that individuals with defective innate immune systems may be more susceptible to pulmonary neoplasia.
Inducible Nitric Oxide Synthase (iNOS), Arginase, and Macrophage Phenotypes
As mentioned earlier, the phenotypes of macrophages in cancer are quite diverse and will not be discussed here in detail. However, we refer the readers to several excellent reviews [152, 191–194]. Nitric oxide synthases can convert arginine to nitric oxide (NO) and citrulline, while arginases convert arginine to ornithine and urea [152]. Three isoforms of NOS exist, however, inducible NOS [195] is the isoform most well studied in inflammation [196]. iNOS is considered a marker for M1 macrophages. High NO concentrations are typically considered detrimental due to the reaction with superoxide to generate peroxynitrite, a free radical [197]. Both iNOS and exhaled NO levels were elevated in NSCLC patients, which supports NO involvement in pulmonary neoplasia [198]. Urethane-induced carcinogenesis in iNOS (Nos2)-deficient mice elicited an 81 % decrease in lung tumor multiplicity compared to wt mice [199]. Reduced angiogenesis was observed in tumors from iNOS-deficient mice measured by vascular endothelial growth factor (VEGF) content and may account for some of the reduction in tumor number [199].
M2 alternative activation by IL4 and IL13 results in increased Th2 responses and increased proliferation, or tumor promotion [193, 194]. Il4 receptor α-deficient mice (Il4Rα −/−) in response to urethane-induced carcinogenesis, developed smaller tumors than those observed in the wild-type BALB/cJ mice [186]. Phenotying the macrophages in urethane-exposed lungs revealed that M2 macrophages predominate in the pre-malignant lesions. In contrast, those lungs bearing carcinomas contain only M1 macrophages. Bone marrow monocytes adopted these expression patterns before entering the circulation. These different phenotypes of macrophages are likely involved in regulating the Th1 versus Th2 cytokine response in the tumor microenvironment and appear to influence tumor development, as was seen in the Ifnγ− and IL4Rα-deficient mouse models [186]. Because of the bone marrow involvement, these two cytokine/cytokine receptors may be early biomarkers for pulmonary AC.
Preclinical Models: Summary
Animal models consistently demonstrate that certain inflammatory pathways are clearly important in lung carcinogenesis . It is true that for some genes, the responses are model specific, e.g., NRF2 in urethane versus MCA/BHT model. Differences between these many models may reflect differences in metabolism, strain, gender, or timing of response, i.e., not all models assess primary tumors, some are metastastic, and some are already initiated (e.g., Kras G12D). Finally, Table 4.1 demonstrates what we currently hypothesize about specific inflammation pathways based on these mouse cancer models, including some of the cytokines/chemokines elevated in these models. Many of these mediators are linked to multiple stages (e.g., NRF2, PPARγ, NFκB), and some are linked to both pro- and anti-inflammatory pathways depending on the stage (e.g., NRF2, PPARγ). This emphasizes the importance of a stage-dependent approach to therapy. Early-stage treatment will likely not be as effective in late-stage treatment when approaching inflammation as a target. As a result, it is sensible to use caution. For example, NRF-2 activators such as sulforaphan have been suggested as a therapeutic approach to lung cancer, however, based on these mouse models and the human data implicating NRF2 as more of an “oncogene-like” transcription factor early in lung cancer [200], clinicians should be somewhat hesitant in this approach. Other pathways, such as innate immune pathways (TLR4 and IFNg), appear counterintuitive to the expected response and yet empirical evidence, both epidemiology and in vivo, supports these pathways as more “anti-inflammatory” in the context of lung cancer. The mechanisms driving these peculiar responses are not clear, but may involve specific subsets of macrophages and lymphocyte populations. The more recent observation of the importance of IL17 in some of these models provides a demonstration of specific subsets of lymphocytes that may drive some of these responses in certain models, but not others (i.e., NTHi and the Kras model).
Preclinical Chemoprevention
Overview
Many preclinical models have been evaluated for multiple inflammation pathways using pharmacological inhibition or activation in animal models of lung cancer to provide further evidence of the importance of specific key pathways . The major pathways tested have been the COX pathways using NSAIDS, lipoxygenase inhibitors to inhibit LOX or enzymes in the leukotriene pathway, prostacyclin agonists, PPARγ agonists, and several different steroids as general inhibitors of inflammation. Some of these studies have led to clinical trials , such as the prostacyclin analog iloprost , however, most have not. While many of these studies have shown some reduction in tumor multiplicity, few demonstrate reductions in tumor incidence. Those that do are dually noted. The majority of these studies were done by providing mouse diet-containing drugs prior to the administration of the carcinogen or directly after to test the effects on promotion . However, differences in dosing, strains, gender, and carcinogen need to be considered when comparing different studies and drugs used .
Glucocorticoids (GC) in Lung Cancer
GCs exert their biological effects primarily through nuclear receptor-dependent regulation of gene transcription [201, 202]. Synthetic GCs suppress a number of immune and inflammatory-mediated responses and are therefore used for treatment of chronic inflammation [203, 204]. They inhibit the expression of pro-inflammatory chemokines, cytokines, adhesion molecules, and enzymes such as COX and iNOS [203–205]. Upon binding to GCs, their receptors release from an inactive complex in the cytoplasm which can then facilitate nuclear translocation, dimerization, and subsequent modulation of gene expression [203, 204, 206]. Activation or repression of transcription of target genes then occurs by the GC-receptor complex binding glucocorticoid responsive elements (GREs) on DNA [206–209]. The GC receptor can alternatively interfere with signaling pathways through interaction with AP-1 or NFκB transcription factors [203, 204, 210, 211]. Several pro-inflammatory mediators are regulated in this manner [204, 205].
Due to the ability to suppress a number of inflammatory indices, GCs have been evaluated as chemopreventive agents in various mouse models of lung carcinogenesis (Table 4.2) [212–221]. The majority of these studies were successful in that they demonstrated some reduction in tumor multiplicity and in a few, tumor incidence. The most efficacious studies were those using aerosolized GCs, namely budesonide. The benefits of targeted delivery to lung are clear including lower doses required, since there are many known adverse systemic side effects of steroids. Equivalent doses of budesonide used for severe asthma patients (5.7–8.6 µg/kg body weight) inhibited tumor formation by 34–60 % [222]; addition of myoinositol elicited further reductions (79 %) [223]. Myoinositol is a precursor to the phosphotidyinositol cycle and a glucose isomer that is involved in Ca+ 2 regulation [224].
Table 4.2
Preclinical studies in mice for both SCC and ADC
Drug | Carcinogen | Strain | Sex | Effect on lung tumor incidence (TI) and/or Multiplicity (TM) | Ref. |
---|---|---|---|---|---|
Glucocorticoids | |||||
Dexamethasone | BaP | A/J | Female | 0.5 mg/kg diet alone reduced TM by 56 %; in combination with myo-inositol (1 %), TM was inhibited by 86 % | 219 |
Cigarette smoke | A/J | Male | Dexamethasone (0.5 mg/kg) and myoinositol (10 g/kg diet) reduced TI and decreased TM by 50 % | 224 | |
Cigarette smoke | A/J | Male | 0.5 mg/kg diet; reduced TM by 64 % | 220 | |
NNK | A/J | Female | 0.5 mg/kg diet alone reduced TM by 41 %; in combination with myo-inositol (1 %), TM was inhibited by 71 % | 219 | |
NNK | A/J | Male | Dexamethasone (0.5 mg/kg diet) and myo-inositol (10 g/kg diet) inhibited TM by 86 % and TM > 1 mm in size by 100 % | 224 | |
NNK | A/J | Male | 0.5 mg/kg diet inhibited TM by 38 % | 220 | |
NNK | FVB | NS | Myo-inositol (10 g/kg diet) and dexamethasone (0.5 mg/kg) inhibited TM in p53 wild-type and mutant strains by ~ 70 % | 223 | |
VC | A/J | Female | 100 μg/kg diet, reduced TM by ~ 45–48 % | 216 | |
Budesonide | BaP | A/J | Female | Aerosolized budesonide (23–126 μg/kg); 83–89 % inhibition of TI, respectively | 226 |
BaP | A/J | NS | 1.5 mg/kg diet; reduced TM by 69.8 %, reduced TS by 94 % | 221 | |
BaP | A/J | Female | Aerosolized budesonide (10 μg/kg, 25 μg/kg) reduced TM by 34 and 60 %; in combination with myo-inositol (3 % diet), additive effects on TM (60 and 79 % inhibition, respectively) | 225 | |
VC | A/J | Female | Dose-dependent (25.1–82.2 %) reduction in TM when fed at 0.6–2.4 mg/kg diet | 218 | |
VC | A/J | Female | Coadministration of budesonide with Zarnestrac (100 mg/kg) reduced TM by 35 and 61 % after, respectively 36 weeks; lower combined doses were as effective as budesonide alone | 222 | |
Beclomethasone Dipropionate | BaP | A/J | Female | Aerosolized (4.8–30 μg/kg body weight) reduced TM by 25–60 % | 225 |
Nonsteroidal anti-inflammatory drugs (NSAIDS) | |||||
Aspirin | BaP | A/HeJ | Female | 50 mg/kg IP; no effect on TM | 232 |
Cigarette smoke | A/J | Male | 300 mg/kg diet; no effect on TM or size | 224 | |
NNK (chr)b | A/J | Female | ASA (294 mg/kg) inhibited TM by ~ 60 % | 233 | |
NNK (chr) | A/J | Female | 588 or 294 mg/kg diet inhibited TM by 53 and 45 %, respectively | 238 | |
NNK | A/J | Male | 300 mg/kg diet had no effect on TM except reduced multiplicity of tumors > 1mm in diameter | 224 | |
MCA + BHT | BALBa | Male | 400 mg/kg diet; no effect on TM, increased tumor size by 17 % | 235 | |
Indomethacin | U | A/J | Female | 0.4 mg/kg diet; reduced TM by ~ 30 % | 236 |
Naproxen | NNK (chr) | A/J | Female | 230 mg/kg diet; no effect on TM | 234 |
Ibuprofen | NNK (chr) | A/J | Female | Reduced TM by 38 % | 234 |
Sulindac | NNK (chr) | A/J | Female | 123 mg/kg inhibited TM by 52 % | 233 |
NNK (chr) | A/J | Female | 123 mg/kg inhibited TM by 53 % | 237 | |
NNK (chr) | A/J | Female | 123 mg/kg inhibited TM by 51 % when fed prior to and throughout the study. Less effective (28 % inhibition) when given before and during NNK; not effective when initiated post-NNK treatment | 234 | |
COX-2 specific inhibitors | |||||
NS398 | NNK (chr) | A/J | Female | 7 mg/kg diet; decreased TM by 35 % | 238 |
Celecoxib | MCA + BHT | BALBa | Male | 500 mg/kg diet; no effect on TM, increased tumor size by 40 % | 241 |
U | A/J | Male | 500 mg/kg diet; no effect on TM or size | 241 | |
Piroxicam | NNK (chr) | A/J | NS | 25 mg/kg diet reduced TM by 30 % | 234 |
VC | A/J | Female | 75 mg/kg diet reduced TM by ~ 50 % | 216 | |
Lipoxygenase Inhibitors | |||||
Nodihydroguaiaretic Acid | U | A/J | NS | 0.1 % in drinking water; reduced TM by 27.8 % | 244 |
A79175 | NNK (chr) | A/J | Female | Reduced TM by 75.5 % | 245 |
Accolate | VC | A/J | Female | 540 mg/kg diet; reduced TM by 29.5 %; decreased tumor size and progression | 246 |
MK-886 | VC | A/J | Female | 30 mg/kg diet reduced TM by 37.8 %; decreased tumor size, and progression | 246 |
S-29606 | BaP | A/J | Female | Nose-only inhalation (0.22, 0.44 mg/kg). 30 and 40 % decrease in TM for the low and high dose, respectively | 247 |
S-30621 | BaP | A/J | Female | Nose-only inhalation (0.22, 0.44 mg/kg). 30 and 40 % decrease in TM for the low and high dose, respectively | 247 |
Zileuton | VC | A/J | Female | 1200 mg/kg diet; reduced TM by 28.1 %, decreased tumor size and progression | 246 |
BaP | A/J | Female | 245.3 mg/kg diet, no effect on TM | 247 | |
PPARγ agonists | |||||
Rosiglitazone | NNK (ip) | A/J | Female | 10 mg/kg ip (3X per week); reduced TM | 255 |
NNK (ip) | A/J | Female | 10 mg/kg ip RGZ, 2.5 mg/kg hydralazine ip., sodium phenylbutyrate 300 mg/kg ip, all dosed 3X per week, selenium, 16 ppm drinking water; reduced TS | 255 | |
Pioglitazone | VC | p53wt/Ala135Val | Female | 15 mg/kg gavage daily for 12 weeks; started 8 weeks after VC; No change TM; ~ 50 reduction in TS in wt and p53 mutant mice | 257 |
NTCU (SCC) | NIH Swiss Webster | Female | 15 mg/kg gavage daily for 24 weeks; started 8 weeks after NTCU; reduced SCC by 35 % | 257 | |
BaP | A/J | Female | 10 mg/kg gavage 2 weeks after BaP for 20 weeks. Reduced TM 5.8 %; Reduced TS 63% | 256 | |
BaP | A/J | Female | 10 mg/kg gavage 2 weeks after BaP and aerosolized budesonide (2.25 mg/ml) for 20 weeks. Reduced TM 55.5 %, same as budesonide alone; TS reduced ~ 10 % more than budesonide alone | 256 |
GCs have additional effects, such as cell cycle regulation; induction of alveolar type II cell differentiation [225, 226], and reduced proliferation of bronchial and alveolar epithelial cells [217, 227]. Budesonide elicits growth inhibitory properties in tumor tissue [217], potentially through induction of growth arrest and/or activation of apoptotic pathways [221]. However, the association between inflammation and cell cycle indices renders it difficult to evaluate the independent contribution of each to tumor suppression, suggesting these drugs may have chemopreventive effects at very early stages of tumor development [228].
Nonsteroidal Anti-Inflammatory Drugs (NSAIDS)
Drugs in this category vary greatly with specificity to either both COX-1 and COX-2 enzymes, or only COX-2. Many studies have been done and the results are inconsistent (Table 4.2) [48, 214, 220, 229–235]. NNK was used for most studies in a chronic design, administered in drinking water. Mice were treated with NSAIDs 2 weeks prior to NNK administration and continued throughout the course of the study. Reductions in tumor multiplicity ranged from 30 to 88 % to that of controls, with one exception [231]. Some of these NSAIDs were found to inhibit NNK bioactivation by p450 or COX isozymes, thus the relative sensitivity of this model may be limited [231, 235]. When different mouse models were used to initiate carcinogenesis, less conclusive results were observed; some NSAIDs reduced [220, 233] while others had no effect [220, 229, 232] on tumor multiplicity. Sulindac sulfone (Exisulind), a metabolite of sulindac that does not inhibit cyclooxygenase, was found to decrease tumor incidence, however, it is likely not through an anti-inflammatory mechanism [236].
Although much attention has been focused on a promotional role of COX-derived PGE2, this does not consistently correlate to tumorigenesis in murine models of lung cancer. Plasma levels of PGE2 were found to positively correlate to tumor multiplicity in one study [235], while two other studies found no predictive value of PGE2 levels on tumor growth [237, 238]. From these studies, it appears that pharmacological inhibition of COX enzymes does not strongly predict reduced tumorigenesis. This may be related to concomitant reductions in antitumorigenic PGI2 [95, 96] and/or enhanced production of leukotrienes [239] when COX1 and 2 activity is blocked.
5-Lipoxygenase Inhibitors
5-LO, one of the alternative pathways in AA metabolism, produces the leukotrienes LTA4, LTB4, LTC4, LTD4, and LTE4 [76]. Leukotriene pathway inhibitors reduce lung cancer cell growth [240] and enhance apoptotic cell death. [240, 241]. 5-LO inhibitors reduced tumor multiplicity regardless of the carcinogen used in murine models of lung cancer (Table 4.2) [241–244]. Leukotriene pathway inhibitors were administered following carcinogen treatment in most studies, indicating an anti-promotional role for these compounds. However, hepatoxicity may limit the effectiveness of some 5-LO inhibitors as therapeutic drugs in humans [245]. A lung cancer chemoprevention trial evaluating the 5LO inhibitor zileuton was initiated but never completed.
Prostacyclin Agonist (Iloprost)
The positive findings with PGIS overexpression inhibiting lung tumor incidence and multiplicity in a variety of models led to an evaluation of the oral prostacyclin analog, Iloprost . FVB/N mice were given oral iloprost at two different timepoints, 1 week prior to urethane and 5 weeks after urethane. Both experimental groups exhibited a decrease in tumor multiplicity compared to mice receiving control chow [97]. These positive results led to a human trial in which high risk current and former smokers received oral iloprost for 6 months. Former smokers treated with iloprost showed significant improvement in endobronchial dysplasia [246], suggesting prostacyclin supplementation could chemoprevent the progression of dysplastic lesions to invasive cancer. Inhalational iloprost studies have been proposed.
PPARγ Agonists
There are several drugs that act as synthetic ligands for PPARγ. The most common are the thiazolidinediones (TZD) class of drugs, such as troglitazide, pioglitazone (PGZ), and rosiglitazone (RGZ). Only pioglitazone is still used clinically for type II diabetes due to its ability to reverse insulin resistance [247]. Some NSAIDS, such as sulindac sulfide [248], can also signal through PPARγ, and this may account for COX-independent effects of NSAIDS in lung cancer growth. Numerous studies have now used several of these PPARγ agonists based on findings that PPARγ induces antiproliferative as well as pro-differentiation properties in lung cells [101]. PPARγ expression was also higher in NSCLC tumors versus the surrounding tissue, although the type of tumor was not assessed [249]. Xenografts of A549 cells to SCID mice treated with TZD or PZD were growth inhibited by 66 % [249]. A549 cells treated with both RZD and gefitinib (an EGFR inhibitor) demonstrated an increase in gefitinibs effects through increased phosphatase and tensin homologue (PTEN) expression [250]. PTEN negatively regulates phosphatidylinositol 3-kinase, involved in cell survival and proliferation [251].
In mice treated with NNK (ip) in the presence or absence of RZD, adenomas were significantly reduced [252] (Table 4.2). Ki67 (a marker of proliferation) was also reduced in RZD-treated mice. Additionally, RZD in the presence of histone deacetylase (HDAC) inhibitors (valproic acid and phenylbutyrate) in the same NNK model also significantly reduced tumor size [252]. Two others studies used PZD to study the therapeutic effects in mice [39, 253]. Vinyl carbamate, a metabolite of urethane (ethyl carbamate), was used to induce ADC followed by treatment with PZD (oral gavage daily, up to 20 weeks) started 8 weeks after carcinogen to assure small adenomas were already present (~ 8 per mouse) [253] (see Table 4.2). Little change in tumor multiplicity was observed, however a ~ 50 % significant reduction in tumor load (size) was seen in this ADC model. The NTCU SCC model was also assessed using a similar protocol and PZD started 8 weeks after NTCU, except PZD was continued for 24 weeks [253]. SCC was reduced by 35 % following PZD treatment compared to untreated mice. PZD was also found to act similarly using benzo[a]pyrene (BaP) as the carcinogen with ~ 63 % reduction in tumor size but little change in multiplicity [39]. When PZD and budesonide were combined following treatment with BaP, there was no change in multiplicity compared to budesonide alone, however tumor load (size) was further reduced by ~ 10 % compared to budesonide alone [39]. These studies demonstrate that activating the PPARγ pathway in conjunction with additional combinations of treatment may provide further reductions in tumor size and development depending on the type of lung cancer, ADC versus SCC.
Preclinical Testing in Animal Models: Summary
Preclinical testing in animal models is an important component of testing specific compounds for toxicity and efficacy prior to considering clinical trials . These early animal studies often identify systemic problems, such as the cardiac effects of COX-2-specific inhibitors (e.g., rofecoxib) [254, 255]. There are several models that have been used in the above described studies, including MCA/BHT, urethane, NTCU, benzo[a]pyrene, and NNK. As discussed earlier, some of these models test complete carcinogenesis, and some test promotion . It may be these differences that account for variability in responsiveness to the test drugs/compounds (e.g., aspirin). The stage of carcinogenesis targeted is important in both these preclinical and clinical studies, because stage-specific effects may occur, as will be discussed below. That is to say some of these chemopreventive drugs may prove useful only prior to developing the tumor (thus during initiation or promotion) while others may only reduce tumor size or decrease metastasis (see below Clinical Trial Overview). Some of the pathways evaluated in mice have demonstrated distinct differences in response, depending on the stage (e.g., NRF2). Anti-inflammatory therapies that have demonstrated some success in human lung cancer are aspirin, inhaled steroids, and oral iloprost, although more clinical trials are needed. PPARγ agonists show therapeutic potential and have demonstrated either reduction in tumor multiplicity or size, thus future studies are warranted. However, some dual anti- and pro-inflammatory activities of PPARγ may limit the utility to specific stages. An additional pathway of interest is blockade of IL17. One small study has been done using IL17 antibodies, thus additional preclinical testing would help inform future clinical trials. As always, one caveat to these preclinical studies is that blockade or activation of inflammation pathways can cause deleterious effects and these effects may not be observed in animal studies compared to human clinical trials . Additionally, species differences in metabolism, gene expression, etc., may influence responsiveness to these pathways of interest.
Clinical Trials
Overview
A variety of clinical trials can provide information to support strategies for chemoprevention of lung cancer [256]. The most reliable are Phase III trials with either lung cancer incidence or lung cancer death as the primary outcome. Due to the long premalignant and preclinical phases of lung carcinogenesis, chemoprevention trials likely will require years of treatment and at least 5–10 years of follow-up to demonstrate an effect. For this reason, secondary endpoints have been studied. The higher the risk in the study population, the more likely a trial is to yield significant results. Individuals with a yearly risk of lung cancer of 1–2 % can be readily identified on the basis of smoking history, presence of airflow obstruction or emphysema, and family history; those with a previous aerodigestive cancer have even higher rates of second primary lung cancer [10]. Large Phase III trials of beta carotene, 13 cis retinoic acid, N-acetyl cysteine, selenium, multivitamin, and mineral supplementation with lung cancer incidence as the primary endpoint have been reported [23, 257–261]. Antioxidant activity was one of the putative chemopreventive mechanisms hypothesized for these interventions. None of these has been positive and beta carotene supplementation increases the risk of lung cancer, particularly in current smokers [24].
Before new agents are considered for Phase III trials of lung cancer chemoprevention , extensive preliminary evidence of efficacy is required [262]. This evidence can include epidemiologic observations, observational evidence from clinical trials with endpoints other than lung cancer, preclinical studies in animal models (see above), and early phase (Phases I and II) clinical trials. Phase I trials usually have endpoints related to tolerability and compliance, both critical for chemoprevention, where a significant number of subjects must undergo treatment to prevent a smaller number of cancers. Phase II trials evaluate intermediate endpoints which ideally should be associated with lung cancer, involved in the disease process and be therapeutically modulated in coordination with the true endpoint (lung cancer). As no chemoprevention agents have proven efficacy for lung cancer, the last criterion currently cannot be met [263]. Phase II trials have utilized airway epithelial dysplasia, epithelial proliferation, and lung nodules as intermediate endpoints. It needs to be emphasized that all of these have been chosen based on biological plausibility and that none are validated as predicting efficacy.
Almost all chemopreventive agents that have been evaluated in clinical trials have some component of anti-inflammatory activity. The two major classes that have received attention are corticosteroids and drugs that alter eicosanoid metabolism.
Corticosteroids
Both systemic and inhaled corticosteroids have shown chemopreventive efficacy for lung cancer in preclinical models [222]. Systemic corticosteroids have too many adverse effects to be considered for lung cancer chemoprevention. Inhaled corticosteroids are commonly used for the treatment of chronic obstructive lung disease and would be attractive for lung cancer chemoprevention . No Phase III trials of inhaled steroids with lung cancer as the primary endpoint have been carried out. Phase II trials of inhaled budesonide and fluticasone with the primary endpoint of airway dysplasia were negative [264, 265]. In the budesonide trial, a secondary endpoint of pulmonary nodule size was assessed by CT scan [265]. It was reported that pulmonary nodules resolved more frequently in the inhaled budesonide group. However, two subsequent Phase II trials of inhaled corticosteroids with pulmonary nodules as the primary endpoint were negative [266, 267]. Several observational studies have suggested that inhaled corticosteroids may have a chemopreventive effect. Parimon and colleagues reported a cohort study of Department of Veterans Affairs patients being treated with high-dose inhaled corticosteroids and exhibiting good compliance [228]. The inhaled corticosteroid group had a reduced lung cancer risk (HR = 0.47; 95 % CI 0.16–0.96) compared to controls. A number of interventional trials assessing outcomes related to chronic obstructive lung disease have been carried out [264, 268, 269]. None of these trials demonstrated a lowering of lung cancer incidence in the inhaled corticosteroid group. An unpublished meta-analysis of these trials (D. Sin, personal communication) demonstrated a trend towards chemoprevention by inhaled corticosteroid (HR = 0.47; 95 % CI 0.22–1.00). Of note, these interventional trials were designed to assess endpoints other than lung cancer and therefore had only a mean observation period of 26 months. While no Phase III trial with lung cancer incidence as the primary endpoint has been carried out, the sum of preclinical and observational evidence suggests that inhaled corticosteroids may have a chemopreventive effect and a definitive, longer duration trials should be considered [270].
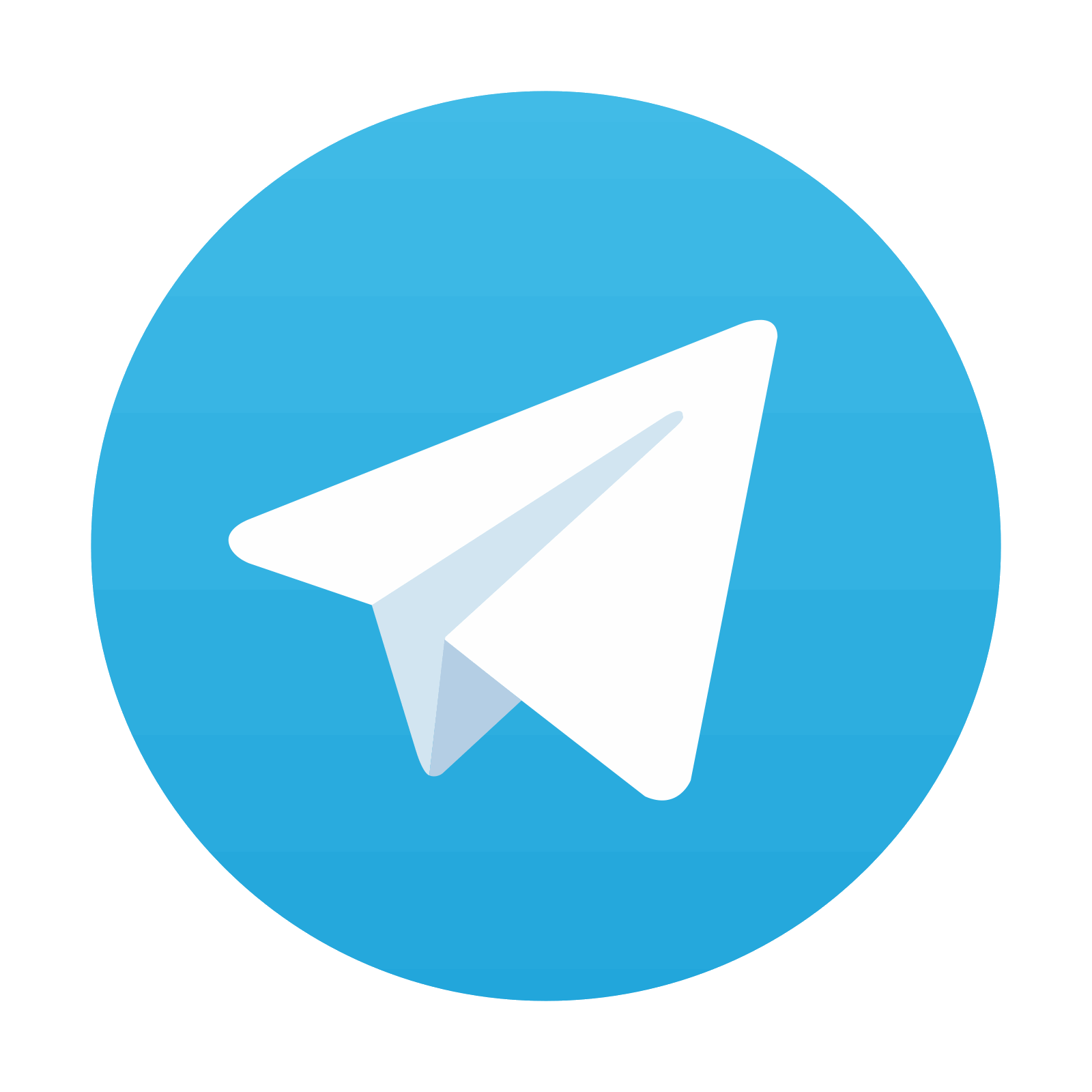
Stay updated, free articles. Join our Telegram channel
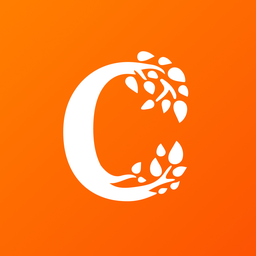
Full access? Get Clinical Tree
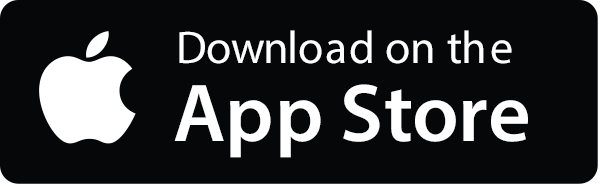
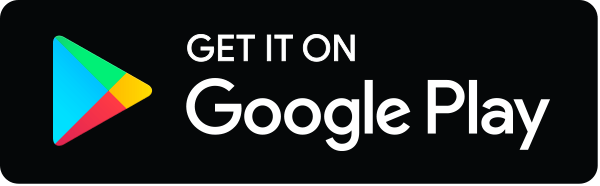