(1)
Department of Cardiothoracic, Transplantation and Vascular Surgery, Leibniz Research Laboratories for Biotechnology and Artificial Organs, REBIRTH – Center for Regenerative Medicine (OE 6217), Hannover Medical School, Carl-Neuberg-Str. 1, HBZ – building J11, H0, 30625 Hannover, Germany
Abstract
The conversion of somatic cells into so called induced pluripotent stem cells (iPSCs) is generally considered as major breakthrough in stem cell research, and cardiomyocytes derived from human iPS cells are considered as promising cell source for disease modelling, drug screening and safety pharmacology, and future therapeutic applications.
This review gives an overview on the molecular basis of cardiac development, recent progress in directing differentiation of pluripotent stem cells into the cardiac lineage and characterisation of the resulting myocytes. Moreover, generation of cardiac cells from disease-specific iPSCs and application of iPSC-derived myocytes for engineering of functional heart tissue and heart repair is discussed.
Introduction
The intrinsic regenerative capacity of the human heart is very limited. Adult cardiomyocytes (CMs) have almost lost their potential for proliferation and cannot be expanded in culture. For many years, the lack of human myocytes represented a major obstacle in basic research in cardiology, in drug screening and safety pharmacology, and certainly also for development of cellular therapies and in vitro engineering of functional heart tissue.
Therefore, cardiomyocytes derived from human pluripotent stem cells (hPSCs) and especially from induced pluripotent stem cells (hiPSCs) hold great promise as a suitable cell source. Since the first report of cardiomyocyte generation from human embryonic stem cells (hESCs) (Kehat et al. 2001) much effort was put into establishing well defined conditions for more reliable and efficient differentiation protocols as well as selection procedures to provide highly enriched cell populations. The detailed molecular and functional characterization of the hPSC-derived cardiomyocytes demonstrated the parallel development of atrial, ventricular and pacemaker cells showing chronotrophic responses to cardioactive drugs, typically with a rather fetal than adult phenotype. In the following we provide an overview on developmental findings that provided (and still provide) important cues for targeted differentiation of pluripotent stem cells into cardiomyocytes and their specific sublineages, on established protocols for improved cardiac differentiation and maturation, and on different genetic and non-genetic strategies for enrichment of hPSC-derived cardiomyocytes. Furthermore, published data on the detailed molecular, structural and functional characteristics of hPSC-derived cardiomyocytes, and their suitability for drug screening and safety pharmacology, as well as first results of their application in disease modelling, tissue engineering and animal models are discussed.
Cardiomyocyte Formation During Heart Development
The development of the vertebrate heart has become more and more complex throughout the evolution. Nevertheless, basic mechanisms of heart development are conserved, and knowledge from different model organisms including zebrafish, xenopus leavis and mice critically contributes to the current understanding of human heart formation.
The major stages of mouse heart development are defined based on morphological and functional aspects and can be further characterized by the expression of specific transcription factors (Bruneau 2002). In a first step, heart development is initiated through the migration of cells from the primitive streak to the anterior region of the embryo where the cardiac crescent is formed. These initial cardiac progenitor cells are characterized by expression of the T-box transcription factors brachyury (T) and Eomes followed by the appearance of Flk1 (fetal liver kinase-1) (cardiac mesoderm) (development stage E7.0 in the mouse). These cells give the rise to the cardiac crescent (E7.5–7.75) with the expression of myocardin and Mesp 1 (mesoderm posterior homolog). Mesp 1 induces a complex network of cardiac transcription factors. Subsequently, the linear heart tube is formed (E8.0) by a later stage of cardiac progenitors specified by the expression of GATA4 (GATA binding protein 4) and Mesp 1. Then the heart tube loops (E8.5-E9.5 mouse, ~ d20-d25 human) (Sizarov et al. 2011) and heart cells start to express Nkx 2.5 (NK2 homeobox 5), dHand (heart and neural crest derivatives expressed), Tbx5 (T-box protein 5) and Mef2c (myocyte enhancer factor 2C). This early progenitor cell population which gives rise to the early heart tube represents the primary heart field. Cells from this first heart field contribute to the left ventricle and the atria. Anterior of the linear heart tube a second progenitor cell population forms. Cells from this so called anterior or secondary heart field were first identified by the expression of FGF10 and could be shown to contribute to the cardiac outflow tract. The expression of the LIM homeodomain transcription factor Isl1 (insulin gene enhancer protein) serves also as a marker for the secondary heart field. Isl1 positive cells contribute to the outflow tract, the right ventricle and the atria. A direct regulation of FGF10 transcription by Isl1 could be shown recently (Golzio et al. 2012). The subsequent chamber formation (E10-E12 mouse, ~ d26-38 human) is characterized by the expression of Nkx 2.5, Irx4 (iroquois homeobox 4), Tbx5 and Hand1/2. Whereas the expression of Irx4 is restricted to the ventricular myocardium, Hand1 is predominately expressed in the left and Hand2 in the right ventricle. HEY2 (hairy/enhancer-of-split related with YRPW motif 2) a downstream target of notch signalling reacts as a ventricle specific transcription factor. The atrial cardiomyocytes are characterized by expression of HEY1, GATA4 and TBX5 (Brand 2003).
To enable the complex cardiac development the necessary signalling pathways are well-orchestrated. Positive as well as negative signals from the surrounding tissue are required. The neighbouring endodermal tissue provides positive signalling by members of the bone morphogenetic protein (BMP) family as well as fibroblast growth factors (FGFs). BMP2 and BMP4 contribute to induce the formation of cardiogenic cells from non-precardiac mesoderm, whereas FGF-signalling is responsible for the further proliferation and survival of these cells. For instance a combination of FGF4 and BMP2 could be shown to induce full cardiac differentiation in non-precardiac mesoderm (Brand 2003). In addition studies on Fgf10 and Fgfr2-IIIb mutant mice revealed that both the ligand as well as its receptor are required for normal heart development (Golzio et al. 2012).
WNT signalling provided by the mesoderm itself controls cardiogenesis via positive signals expressed in the precardiac mesoderm. Negative acting signalling molecules of the WNT/ßcatenin pathway, expressed in the cardiogenic mesoderm, have to be repressed by inhibitors like dickkopf (Dkk-1) to support early cardiogenesis (Burridge et al. 2012).
Initiated through the above extracellular signals and the appearance of various cardiac transcription factors, the increasing expression of different proteins of the contractile apparatus forms the basis for later heart function. Also, the different functional characteristics and distinct contractile properties of the heart chambers are defined by chamber specific structural proteins. The contractile protein myosin consists of two pairs of myosin light chains (MLC) and two heavy chains (MHC). The atrial subtype of myosin light chain (MLC2a) is initially expressed in the linear heart tube and later exclusively in the atrium whereas the ventricular subtype (MLC2v) is solely expressed in ventricular cells throughout development (Small and Krieg 2004). The two different isoforms αMHC and βMHC containing the ATP binding sites constitute the motor function of the myosin molecule, αMHC with high Ca2+ and actin activated ATPase activity results in faster shortening velocity of the cardiac fibers whereas βMHC with lower ATPase activity results in slower shortening velocity (Nadal-Ginard and Mahdavi 1989). In the ventricle of developing mammals ßMHC is the most abundant isoform, however directly before birth, this switches to the αMHC subtype. In small animals αMHC becomes the prevalent isoform in the adult ventricle (>90%), whereas in large mammals including humans the ventricular myocardium is predominantly composed of the slow βMHC isoform (>95%) (Miyata et al. 2000; Narolska et al. 2005). Finally the maturation/septation from E12 till birth (in humans between week 4 and 7) (Lamers and Moorman 2002) is specified by the expression of Nkx 2.5, TBX5, RXRα (retinoid X receptor alpha), FOG-2 (Zfpm2, zinc finger protein, multitype 2), GATA4, TEF-1 (TEA domain family member 1) and Sox4 (SRY-box containing gene 4). For establishing the left right identity of the atria the expression of pitx2 (paired-like homeodomain transcription factor 2) is required (Bruneau 2002). Septation of cardiac chambers and therefore the unidirectional bloodflow is ensured by functional cardiac valves. Signals between myocardium and endocardium mediated by BMP2, TBX3, TGFß, and the NOTCH pathway are necessary for correct positioning and function of the cardiac valves (Srivastava 2006). During mouse development these events take place during the first 12 days after fertilization whereas human development generally takes longer and cardiac valves are formed after 35 days (Sizarov et al. 2011). Even before this complex development is completed the primitive tubular heart begins to beat in mouse on day 8 and in human about 3 weeks after fertilization (Brand 2003).
During fetal development cardiomyocytes rapidly divide but exit the cell cycle postnatally. The majority of terminal differentiated cardiomyocytes do not reenter the cell cycle therefore an increase in cell size (hypertrophy) is responsible for increasing cardiac mass after birth (Burridge et al. 2012). Due to this limited proliferation potential adult cardiomyocytes cannot be expanded in culture. The opportunity to generate virtually unlimited numbers of CMs differentiated from pluripotent stem cells might facilitate various applications of these cells.
Differentiation of Pluripotent Stem Cells Towards Cardiomyocytes
The first in vitro differentiation approach that resulted in spontaneously contracting cardiomyocytes was developed by A. Wobus and co-workers using murine embryonic stem cells (Wobus et al. 1991). After removal of feeder cells that support the maintenance of an undifferentiated state, cells were aggregated to form so called embryoid bodies (EBs). Within such EBs, the stem cells developed into various differentiated lineages mimicking at least in part the developmental processes that occur in a normal embryo. Since EB-based differentiation typically results in formation of a variety of different cell lineages, this approach is frequently considered as non-targeted or random differentiation. However, strictly speaking this is not correct. EB-based protocols are typically based on culture media contain fetal calf serum, which provides lot-dependent combination of cytokines and other differentiation-modulating compounds. Moreover, EBs consist of more or less organised tissue structures with distinct cell types that provide molecular signals for differentiation to neighbouring cells. For instance, EBs typically contain a surrounding cell layer of endodermal cells. Although not as well orchestrated as in a native embryo, molecular cues provided by a certain tissue component such as early endoderm result in specific differentiation pathways in individual cells of the EB.
More recently, EB-based differentiation protocols have been adapted to human ES cells (Kehat et al. 2001). Also human iPS cells have been differentiated into beating cells with phenotypic properties of functional cardiomyocytes (Haase et al. 2009; Zhang et al. 2009).
Typically, efficiencies of EB-based cardiac differentiation are relatively low, however, using pluripotent stem cell lines such as the hESC line (H9.2) or HES-3, efficiencies between 8 and 70% of beating EBs, which probably correspond to a few percent of myocytes, only, were obtained (Xu et al. 2002). In the meantime, these rather inefficient and barely reproducible approaches could be optimized by utilizing molecular insights from mouse development.
As already discussed above, developmental studies demonstrated that surrounding endodermal cells provide required inductive as well as inhibitory signals for the cardiac differentiation of pluripotent cells. Consequently, it was investigated whether endodermal cells can induce cardiogenesis in embryonic stem cells in vitro, also, and a mouse cell line (END-2) that resembles visceral endodermal cells was shown to induce cardiogenesis in mouse P19 and murine embryonic stem cells (Mummery et al. 1991). More recently, this finding could be transferred to human cells demonstrating induction of cardiogenesis in hESCs after co-culture with END-2 cells. Notably, different hESC lines could be differentiated to the cardiomyocyte lineage even if a standard EB based approach was not successful for generating mesodermal cell types from these specific hESC lines (Mummery et al. 2003). In addition, significant improvement of the protocol could be achieved by applying serum free conditions. Further work demonstrated that the inductive effect of END-2 cells does not depend on direct cell-cell-interaction but depends on soluble factors. Thus, serum free medium conditioned by END-2 cells was able to induce cardiomyogenesis, and depletion of insulin was identified as one underlying mechanism of the observed cardiogenic END-2 effect. On the other hand, insulin depletion alone was insufficient as other cell types with equivalent insulin-depleting activity did not show cardiomyogenic potential. Interestingly, microarray analyses revealed up-regulated expression of enzymes connected to the prostaglandin (PGI2) synthesis in END-2 cells. In fact supplementation of the serum free and insulin free differentiation medium with PGI2 could mimic the effect of END-2 cells or conditioned medium thereof on cardiomyogenesis (Xu et al. 2008a).
In the end, however, all extensive experimental efforts on barely controlled random differentiation, in embryoid bodies and serum-containing media, or in coculture settings, did not lead to efficient and well reproducible protocols.
More recently, groundbreaking substantial work especially of the group of G. Keller (Kattman et al. 2011b) utilized the increasing knowledge on molecular cues and pathways in vertebrate heart development and led to differentiation protocols based on the sequential application of specific inducers for more efficient targeted differentiation.
Notably, development of such protocols and identification of crucial differentiation factors would have been barely possible without the recent advancements of technologies for generation of pluripotent stem cell lines that enable monitoring of cardiac differentiation through integration of transgenic reporters (Elliott et al. 2011). Long-term systematic research using suitable transgenic reporter cell lines step by step deciphered the molecular pathways underlying differentiation (Murry and Keller 2008).
In the meantime, sequential protocols for the efficient induction of cardiomyogenesis in hPSCs have been developed by several groups starting from feeder-based cultures as well as monolayer hPSC cultures. In stepwise approaches, the starting populations were manipulated by adding activators and/or inhibitors of signalling pathways, either as purified or as recombinant proteins, to sequentially form mesendodermal progenitors cells, cardiovascular and cardiac progenitors, and finally cardiomyocytes with typically rather immature phenotype (Burridge et al. 2012). Notably, establishment and optimization of these approaches would not have been possible through the commonly used endpoint analysis of beating cluster and expression of cardiomyocyte specific markers like cardiac troponin T (cTNT) or sarcomeric α actinin. In contrast, the stepwise design required a detailed characterization of the emerging intermediate progenitor populations with respect to lineage-specific cellular marker profiles and suitable molecular tools for their detection. Continued cardiac specification of the respective subpopulations was monitored especially by the appearance of transcription factors such as Mesp 1 and Nkx 2.5 (Kattman et al. 2011b), or by utilizing genetically engineered reporter cell lines which express fluorochromes under the control of a cell-lineage specific promoter, like Nkx 2.5 (Elliott et al. 2011). In combination with developmental data these tools and technologies allowed for targeted manipulation and tight monitoring of the necessary lineage decision during in vitro differentiation of pluripotent stem cells.
As a first step towards targeted generation of cardiomyocytes, induction of mesendoderm formation as visualized by expression of the T-box transcription factor brachyury was achieved by adding a combination of activin A and BMP4 in some cases supported by addition of FGF2 (Murry and Keller 2008; Burridge et al. 2012) Wnt and activin/nodal/TGF-ß signaling block ectoderm formation but induce mesendodermal differentiation. In addition, also BMP-4, has an inhibitory effect on ectoderm formation and supports mesendoderm formation, although apparently not being essential (Murry and Keller 2008). The subsequent formation of cardiac mesoderm with its continued expression of the T-box transcription factor brachyury could be visualized by temporal expression of the surface marker KDR (kinase insert domain receptor (human homolog to FLK-1)) in combination with PDGFR-α (Kattman et al. 2011b). During this 2nd stage of differentiation, activin dose-dependently supports endoderm and mesoderm formation, whereas now inhibition of Wnt-signalling is required for efficient formation of cardiac mesoderm (Murry and Keller 2008; Willems et al. 2011). TGFß/NODAL signalling as another important molecular pathway was modified by NOGGIN (Burridge et al. 2012). Notably, especially effects of activin/nodal/TGF-ß and BMP signaling critically depend on the dosage and timing (Kattman et al. 2011b). Further differentiation of the above specific subpopulations confirmed their cardiomyogenic potential through correlation of the early marker expression with increased cardiomyocyte content (Kattman et al. 2011b) which can be quantified by immunostaining for myocyte-specific structural proteins such as αMHC, cTNT or sarcomeric α actinin (Haase et al. 2009; Zhang et al. 2009). ßMHC can be utilized for detection of more mature human cardiomyocytes (Lundy et al. 2013).
In general, however, the protocols discussed above are typically highly cell line dependent, and time consuming adaption and fine-tuning of concentrations and application time points is necessary to obtain similar results for the cell lines of interest (Kattman et al. 2011a). Even more critical is the fact that the established protocols are based on recombinant/purified proteins. Such proteins are not only very expensive, but considerable lot-to-lot variations prevent the development of highly reproducible and robust differentiation protocols especially for clinically applicable large scale production of myocytes in a commercial scale.
In view of the above significant drawbacks of protein-based induction of differentiation, small chemically synthesized, inductive molecules continuously moved into the focus of stem cell research. Some compounds such as 5-aza-2′-deoxycytidine (5-aza-dC), retinoic acid (RA) or dimethyl sulfoxide (DMSO) have been reported already years ago to promote cardiomyogenesis in murine and human pluripotent stem cells (Xu et al. 2002; Yoon et al. 2006). By utilization of transgenic ES cells (αMHC-GFP) for screening a chemical library Takahashi et al. (2003) were able to show an inductive effect of ascorbic acid on the cardiac differentiation (Takahashi et al. 2003). Notably however, the above compounds presumably act rather unspecific and do obviously not support well orchestrated development of cardiac lineages.
Subsequently, a more selective administration of small molecules could also been shown for different pathways. Graichen et al. (2008) reported a regulating role of the p38 MAP (mitogen-activated protein) kinase pathway, which had also been described to be involved in the regulation of cardiomyocyte mitosis. This pathway could be manipulated by the compound SB203580. Addition of concentrations ≤10 μM during the first 24 h of differentiation lead to a remarkable increase in the number of beating areas and expression of cardiac specific genes in comparison to untreated cultures (Graichen et al. 2008).
Confirming the role of FGF signalling, the FGF receptor inhibitor SU5402 was shown to have a positive effect on the early stages of cardiomyogenesis in combination with BMP2 (Tomescot et al. 2007).
Again taking advantage of transgenic pluripotent reporter cell lines that enable monitoring of cardiac differentiation, more recently novel small compounds were identified that strongly support cardiac differentiation of hES and hiPS cells. By high content screening of two commercial available small molecule libraries on HESCs, which were predifferentiated under mesendoderm inductive conditions, an inhibitor of the canonical WNT pathway (IWR-1) was identified as a potent activator of cardiomyogenesis (Willems et al. 2011). Similar to the biological WNT/ßcatenin inhibitor Dkk-1 other WNT inhibitors (PORCN, 53AH, XAV939) which act on different levels of the same pathway were also able to induce cardiomyogenesis in this predifferentiated cell population (Willems et al. 2011). Based on mouse developmental studies positive BMP signalling and inhibition of WNT signalling promotes mesoderm and cardiac mesoderm formation. Therefore, the combination of BMP4 supplementation with inhibition of the ß-catenin signalling pathway by small molecules (IWR-1 as well as IWP-4) (Ren et al. 2011) could further increase expression of genes described for cardiac mesoderm or cardiac progenitor cells.
Also, replacement of previously identified inductive proteins through known activators and small compound inhibitors of the respective signaling pathways finally led to much more efficient and reproducible differentiation protocols. Subsequently a study by Lian et al. (2013) could demonstrate robust cardiac differentiation by manipulating the canonical WNT signalling by small molecules, only. Applying two small molecules, a Gsk3 inhibitor (CHIR 99021) for activation of the ß-catenin signalling for 24 h (mesoderm induction) and in a second step blocking of WNT signalling to initiate cardiac specification by addition of IWP-4 or IWP-2 in a well defined temporal manner resulted in a highly efficient and quite robust differentiation protocol with cardiomyocyte contents of up to 98% (Lian et al. 2013).
Beside WNT signalling additional pathways (TGFß, BMP and FGF signalling) are involved in cardiomyogenesis. Controlling FGF as well as BMP signalling by small molecules (FGF receptor inhibitor SU5402 and dorsomorphin, respectively) was already been shown to improve cardiomyogenesis. Combining different small molecule based differentiation strategies might lead to even more robust and ideally cell line independent protocols.
The different signalling molecules and addition time points in the course of differentiation are summarized in Fig. 5.1. It is distinguished between growth factor dependent (red) and application of small molecules only (green) to induce mesoderm, for cardiac specification and finally for cardiomyocyte differentiation.
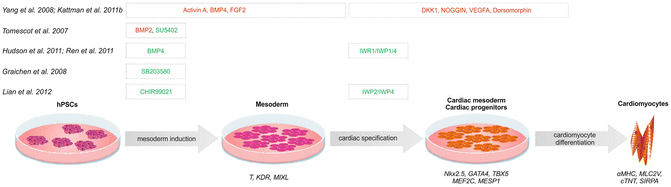
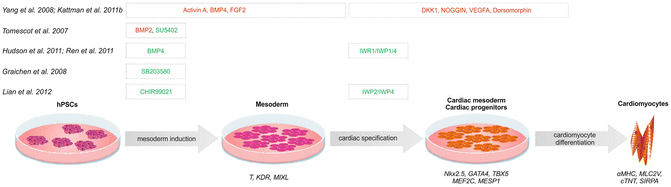
Fig. 5.1
Sequential differentiation protocols leading cells in the desired direction. By addition of growth factors and/or small molecules in a concentration and temporal dependent manner hPSCs are guided to develop into mesodermal cells, cardiac mesoderm, cardiac progenitors and finally cardiomyocytes. Induction and further specification can either be achieved by the use of specific growth factor combinations (red) additionally supported by small molecules or based on the addition of small molecules, only (green). Abbreviations: hPSCs, human pluripotent stem cells; BMP, bone morphogenic protein; FGF2, fibroblast growth factor 2; DKK1, dickkopf homolog 1; NOGGIN, BMP signalling inhibitor; VEGFA, vascular endothelial growth factor A; IWR1, inhibitor of WNT response 1; IWP4, inhibitor of WNT production 4; SU5402, FGF receptor inhibitor; CHIR 99021, inhibitor of Gsk3; SB203580, inhibitor of p38 MAP kinase pathway; T, T-box transcription factor brachyury; KDR, kinase insert domain receptor; MIXL, Mix paired-like homeobox; Nkx2.5, NK2 homeobox 5; GATA4, GATA binding protein 4; TBX5, T-box protein 5; MEF2C, myocyte enhancer factor 2C; MESP1, mesoderm posterior 1 homolog; αMHC, myosin heavy chain 6; MLC2v, myosin light chain 2V; cTNT, cardiac troponin T type 2; SIRPA, signal-regulatory protein alpha
Selection of Generated Cardiomyocytes
Although substantial progress was made concerning the efficiency and reproducibility of differentiation protocols identification and further purification of the desired cell population still appears indispensible, especially as a safety measure to prevent teratoma formation after transplantation of PSC-derived cell preparations.
Different approaches and strategies which take advantage of specific properties of the desired cells are meanwhile available for the selection of cardiomyocytes from mixed populations. One method for the non-genetical selection uses the high mitochondria content of cardiomyocytes compared to other cell types. Staining of differentiation cultures with the non toxic mitochondrial dye tetramethylrhodamine methyl ester perchlorate (TMRM) results in populations with high, intermediate and low fluorescence activity. Cardiomyocytes with high mitochondria content could therefore be isolated from the highly positive population. Utilizing this reversibly labelling of mitochondria, cardiomyocyte populations with purities of up to 99% were reported (Hattori et al. 2010). However, in direct comparison with a common genetic selection strategy the TMRM based purification showed significantly lower purity and very much lower cell yields as the genetic selection approach (Kensah et al. 2012).
Interestingly, Tohyama et al. (2013) also established selection strategies, which take advantage of cell type specific metabolic differences. In contrast to most other differentiated hPSC derivatives, cardiomyocytes are able to metabolise lactate as energy source, and could therefore be enriched in lactate supplemented and glucose depleted medium (Kawamura et al. 2012; Tohyama et al. 2013) Likewise transferring differentiation cultures to hypertonic solutions with osmotic pressure ≥370 mOsm/kg resulted in enrichment of cardiomyocytes.
In the meantime, also surface marker based approaches for enrichment of cardiac progenitors and cardiomyocytes are available. As shown by Kattman et al. (2011a, b) identification of cardiac progenitors by expression of KDRpos/C-KITneg or KDRpos/PDGFR-αpos and further propagation of the respective population under optimal conditions concerning application of growth factors results in an increase of cardiomyocyte content with up to 80% for the KDRpos/PDGFR-αpos fraction (Kattman et al. 2011b).
By extensive screens of known antibody libraries against human cell surface molecules two groups could recently identify markers which allow for sorting more mature cardiomyocytes from differentiation cultures. Uosaki et al. (2011) screened a library consisting of 242 antibodies and could identify vascular cell adhesion molecule 1 (VCAM1) as a cardiac specific surface marker which labelled 80% of the cardiac troponin T positive cells (Uosaki et al. 2011).
Dubois et al. (2011) utilized a Nkx 2.5-eGFP reporter cell line for screening a library of 370 anti CD antibodies and could identify signal regulatory protein alpha (SIRPA) as a marker for cardiomyocytes. Staining against SIRPA and subsequent sorting of positive labelled cells resulted in a 98% cardiac troponin t positive population (Dubois et al. 2011).
Another approach to identify a suitable surface marker used once more insights from the vertebrate heart development. The activated leukocyte cell-adhesion molecule (ALCAM, CD166) a marker with a specific temporal expression in the developing heart could be shown to be co-expressed with early cardiac markers like Nkx 2.5, GATA4, MEF2C and TBX5. 85% of the ALCAM positive population were also positive for αMHC (Rust et al. 2009).
Application of these strategies allows for generation of highly enriched cardiomyocyte populations either by fluorescence activated cell sorting (FACS) or magnet assisted cell separation (MACS).
Due to the initial lack of suitable surface markers for the selection of cardiomyocyte populations classical cardiomyocyte selection strategies were based on stable genetically modified cell lines that carry randomly inserted transgenes mediating an antibiotic resistance (e.g. aminoglycoside phosphotransferase meditating a neomycin resistance or the puromycin acetyl transferase) or the expression of a fluorescence marker (eGFP; mCherry) under the control of a cardiomyocyte type specific promoter. Constructs carrying a resistance gene for neomycin driven by the αMHC promoter were first established for the selection of cardiomyocytes from murine ESCs (Klug et al. 1996). Applying these strategies >99% pure cardiomyocyte populations could be obtained. These approaches were also adapted for the selection of human cardiomyocytes. The construct used for the murine ESCs was also applicable for the selection of cardiomyocytes from hESCs differentiation with purity of up to 99% (Xu et al. 2008b). Beside this first strategy the myosin light chain 2 v promoter (MLC2v) could be utilized for the specific expression of different reporter genes for the selection of cardiomyocytes with similar yields (Anderson et al. 2007; Huber et al. 2007) Notably, in case of random genomic integration of transgenes such genetically engineered cell lines will barely be clinical applicable due to potential insertional mutagenesis. Also, the time consuming generation of these lines, the dependency of expression levels on the genomic integration site and the frequently observed silencing of transgenes limits the usefulness of such lines. Notably, however, efficient site-specific gene editing techniques which allow for safe long term transgene expression at well defined genomic integration sites in hPSCs could recently be developed. Targeted induction of double strand breaks by employing tailored designer nucleases, such as zinc-finger nucleases (ZFN) and transcription activator-like effector nucleases (TALENs) or RNA-mediated editing by CRISPR-Cas leads to enhanced homologous recombination based gene integration in hPSCs with very high efficiencies (Gaj et al. 2013). These techniques facilitate not only highly efficient generation of genetically engineered cell lines with more robust transgene expression and negligible transgene silencing but may also be in accordance to regulatory requirements for clinically applicable cell lines. Since the high purities of up to 100% (Kensah et al. 2012) combined with superior cell vitality that can be achieved through genetic selection procedures could so far not be obtained with any of the non-genetic selection techniques, the novel targeted genome engineering technologies discussed above may represent the most advanced safe, efficient and reliable cardiomyocyte enrichment approaches.
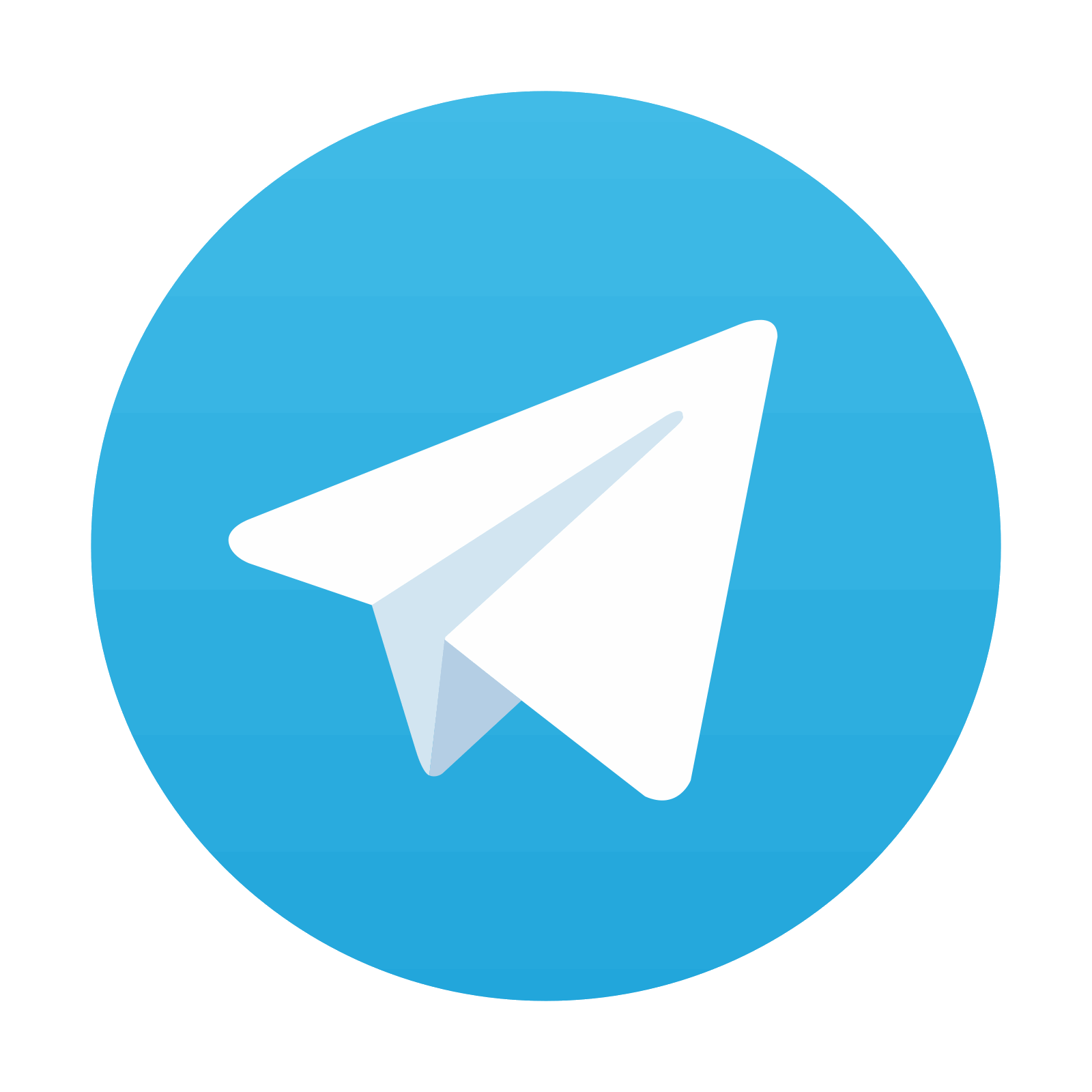
Stay updated, free articles. Join our Telegram channel
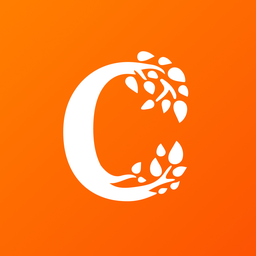
Full access? Get Clinical Tree
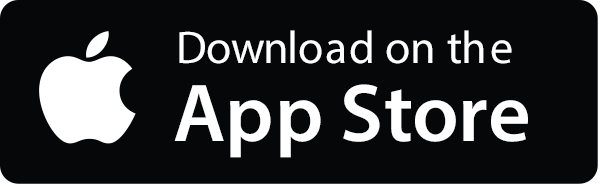
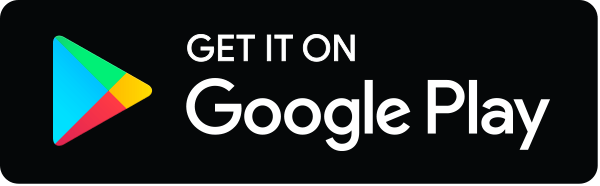