TLR
Ligand/agonist
Potential therapeutic agents
TLR1
Triacylated lipoproteins, lipoteichoic acid, peptidoglycans
PAM3CSK4 [93]
BCG
TLR2
Endogenous DAMPs (HSP, HMGB1, uric acid, fibronectin, ECM proteins) [5]
BCG [97]
HSP60 [98]
TLR3
Viral dsRNA, synthetic analogs of dsRNA
Poly-I:C [99]
Ampligen (AMP-516) [100]
IPH3102 [101]
Poly-A:U [102]
TLR4
LPS, various DAMPs [5]
Eritoran [103]
BCG [97]
TLR5
Bacterial flagellin
Flagellin, CBL502 [104]
TLR6
Diacylated lipopeptides and endogenous DAMPs (HSP, HMGB1, uric acid, fibronectin, ECM proteins)
CBLB613 [107]
TLR7
Viral ssRNA
Imiquimod [108]
Imidazoquinoline 852A [109]
IPH 3201 [110]
SC-1, SC-2 [111]
TLR8
Viral ssRNA
Imiquimod [108]
IPH 3201 [110]
SC-2 [111]
TLR9
BCG
CpG-ODNs [112]
IMO-2055 [113]
ISS1018 [114]
PF-3512676 [115]
17.3 Sarcoma Antigens as Targets for Immunotherapy
Like other cancers, sarcoma cells possess unique tumor antigens that may serve as targets for immunotherapy strategies. Many of the current immunogenic tumor markers have been discovered via serological analysis of recombinant cDNA expression libraries (SEREX). This assay is used to find antigens that have elicited an immune response in their respective hosts [18]. The tumor antigen family of “cancer-testis antigens” (CTAs) was originally discovered using this method and has several notable targets for immunotherapy. Well-known CTAs include NY-ESO-1 and the SSX and MAGE family of proteins [19]. These proteins are grouped together into the subclass of CT-X genes as they are all located on the X chromosome and are preferentially expressed in the testis as opposed to other normal tissues. Importantly, these proteins have been found to be expressed in a variety of malignancies and have been associated with immune reactivity. These characteristics make this family of tumor antigens ideal targets of immune-based therapy, given its limited expression on normal tissues and degree of immune recognition.
17.3.1 NY-ESO-1
One of the best characterized CTAs is NY-ESO-1, a 180 amino acid intracellular protein that also belongs to the CT-X familial subgroup which is normally only expressed on testis tissues [20]. As a CTA it is expressed sporadically on soft tissue sarcomas as well as other malignancies but has a high incidence of expression on synovial sarcomas as well as myxoid/round cell sarcomas [21, 22]. NY-ESO-1 is most frequently expressed heterogeneously within tumors but is known to be highly immunogenic. As evidence of its immunogenicity, NY-ESO-1-specific antibodies are frequently found in patients with corresponding antigen-expressing tumors. In certain cancers it has been observed that as the stage of the disease increases, so does both the frequency and intensity of the immune response to NY-ESO-1. Despite the detection of antibodies, there has been no evidence that the presence of these antibodies confers a survival benefit. However, when antibodies to NY-ESO-1 are detected, a higher likelihood of response to ipilimumab (a CTLA-4-blocking antibody discussed in proceeding sections) has been observed [23]. Whether or not this is due to an enhanced immune response due to ipilimumab or if antibodies to NY-ESO-1 serve as a marker for a response has yet to be determined.
17.3.2 SSX
The pathognomonic characteristic of synovial sarcoma is a specific translocation between SYT (synovial sarcoma translocation) gene, currently also known as SS18 (synovial sarcoma gene of chromosome 18), and SSX (synovial sarcoma X chromosome breakpoint) [24]. There are now over ten known members of the SSX gene family; however, only SSX 1, SSX2, and SSX4 are known to occur in the setting of synovial sarcoma with translocations of SSX 1 and SSX2 representing the majority. This mutation occurs in greater than 95 % of all synovial sarcomas and as such this mutation can be utilized to help confirm the diagnosis [25]. Generally, the last 8 amino acids of SYT are replaced by the 78 amino acids from the C-terminus end of SSX to generate the fusion protein SYT-SSX [26]. While SYT overexpression alone does not lead to tumor formation, this specific combination with SSX does lead to activation and subsequent tumor formation [27]. Unfortunately, expression of SSX is seen intensely in only a subset of tumor cells with the majority of cells having little or no expression. Despite this, given the immunogenicity of SSX, it remains a potential target for immunotherapy.
17.3.3 ALK
Anaplastic lymphoma kinase (ALK) is a receptor tyrosine kinase (RTK) and was first described as an oncogene in 1994 in anaplastic large-cell lymphoma [28]. It has since been found to have a high occurrence rate in inflammatory myofibroblastic tumors (IMTs) as well as sporadic association with other sarcomas including multiple forms of lipomatous tumors/sarcomas, alveolar rhabdomyosarcoma, Ewing sarcoma/primitive neuroectodermal tumors, leiomyosarcomas, and extraskeletal myxoid chondrosarcomas [29]. The relevance of ALK expression in non-IMTs is unclear, but there is an approximately 50 % incidence of clonal rearrangement of the ALK gene in association with IMTs. Given the current enthusiasm for tyrosine kinase inhibitors (TKIs), ALK represents a promising target. The TKI crizotinib, which is a competitive inhibitor of both the ALK and MET tyrosine kinases, has been extensively evaluated in ALK-expressing lung cancer but has also been reported as a therapy for IMT [30]. However, similar to other TKIs, crizotinib treatment resistance appears to be a limiting factor [31].
17.3.4 HHV8
The role of the immune system is clearly evidenced in Kaposi’s sarcoma (KS), a low-grade vascular neoplasm that involves the epithelium. From an epidemiologic standpoint, KS can be grouped into four forms: classic KS in elderly men of European Jewish or Mediterranean descent, endemic KS in Central and Eastern Africa not associated with HIV, iatrogenic KS in the setting of immunosuppression for organ transplantation, and epidemic/AIDS-KS [32]. Human herpesvirus 8 (HHV8), also known as Kaposi’s sarcoma herpesvirus (KSHV), is the causative agent for the development of KS; the rate of HHV8 infection directly correlates to the incidence of KS.
KS tumor cells arise from lymphatic endothelial cells, with HHV8 playing a critical role in the neoplastic transformation of these cells. Several factors are upregulated by HHV8 including vascular endothelial growth factor receptor 3, lymphatic vessel endothelial receptor 1, latency-associated nuclear antigen, and podoplanin [33]. Additionally, activation of the phosphatidylinositol 3-phosphate kinase/Akt/mammalian target of rapamycin pathway also plays a role in development of tumor formation [34]. Despite multiple upregulated genes, HHV8 infection does not usually lead to development of KS in immunocompetent individuals. KS tumor cells are unique from other tumors in that they do not generate tumors in nude mice, nor will they grow in vitro as they require cytokines for continued growth [32]. In addition to these factors, host immunosuppression is a key component to the development of clinical disease.
Initiation of highly active antiretroviral treatment (HAART) for HIV-associated KS or reduction of immunosuppression in organ-transplanted patients can lead to regression of KS, likely by increasing immune system activity against HHV8 but also possibly by reducing HIV-associated inflammation and associated cytokines. Initiation of HAART within the HIV population has been associated with a concurrent decrease in the incidence of KS. Prospective trials of HAART in patients with KS have shown decreases in both HIV and HHV8 viral loads and were subsequently associated with regression of the KS [35, 36]. Recently, a trial of rapamycin in combination with HAART showed some increased effectiveness in inducing a clinical response in KS tumors [34]. The use of antiviral agents, such as ganciclovir, may prevent the development of KS, but specific agents which take advantage of HHV8 once tumors are established are being explored [37].
17.4 Preclinical Models of Immunotherapy for Sarcoma
The ability to treat sarcomas by taking advantage of TLR agonists or immune-targeting of sarcoma-associated antigens has been enhanced by the use of preclinical animal models. Several models exist with distinct advantages and characteristics that allow for the testing of novel immunotherapy strategies. The simplest and classic animal model for sarcoma is using established in vitro sarcoma cell lines and immunocompromised mice. Cells cultivated in vitro could then be transplanted either subcutaneously or implanted as an orthotopic xenograft [38]. While important work has been done using this model, it has inherent flaws as the tumors develop without the selective pressures of an intact host immune system and various forms of immunotherapy are difficult or impossible to evaluate [39]. Additionally, serial passages of tumor cell lines in tissue culture can introduce mutations, favor cells that are capable of growing in vitro, and represent cells from a diverse patient population. Finally, there are a myriad of interactions between the mouse stroma and the human tumor cells that can affect aspects of tumor progression and experimental treatments.
17.4.1 Methylcholanthrene (MCA)
The classic model for spontaneous induction of sarcoma in a murine model is the injection of 3-methylcholanthrene (MCA), first described in 1943 by Gross [40]. Since that time, the complex mechanism of MCA-associated tumorigenesis has been elucidated. The process begins with the binding of MCA to cytochrome P450 and other enzymes [41]. The subsequent metabolites react with guanine, producing DNA adducts that lead to mutations in p53 [42]. MCA also becomes hydroxylated, ultimately forming a reactive carbonium ion that is highly mutagenic and carcinogenic, with these mutations ultimately leading to the development of a spindle cell sarcoma. MCA has been applied to various strains of mice to establish multiple in vitro sarcoma cell lines, thus allowing a homogenous population of tumor cells to be examined repeatedly in vivo. Since its first description, this model has been used extensively to further elucidate properties of soft tissue sarcomas. This model is characterized by the fact that the tumors which develop after administration of MCA are diverse and mimic clinical conditions but may make immunologic experimental design more complicated. For tumor grafts established by injection of an isolated, clonal cell line grown in culture, experimental design may favor immunologic therapy testing but have limited translation clinically.
17.4.2 p53 and Nf1
In an effort to further define the role of p53 in tumorigenesis, chimeric mice for wild-type and null p53 (p53+/−) were generated. When these chimeric mice were crossed, it was found that the majority of mice that were homozygous for mutant p53 developed spontaneous tumors, namely, lymphomas and sarcomas, by 6 months of age [43]. In this model it was noted that common tumors in the original strain of mice (i.e., lymphomas and testicular tumors) developed spontaneously in the p53−/– mice at a much younger age, leading to the conclusion that loss of p53 function accelerates the development of predisposed tumors.
Similarly, a murine model of neurofibromatosis was attempted to be created; however, peripheral nerve sheath tumors were unable to be generated in the heterozygote model using the Nf1 gene. As germ line homozygosity (Nf1−/−) was found to be lethal at day 14 of gestation, a chimeric mouse model was generated that was partially composed of Nf1−/− [44]. These mice were then crossed with the p53 null mice to generate p53−/+; Nf1−/+. Mice that had a loss of heterozygosity (LOH) of only one of these genes survived for about 10 months. However, mice that lacked both functional copies of p53 and Nf1 on chromosome 11 survived for only 5 months and had a much higher predisposition for the development of soft tissue sarcomas. The tumors that develop in these mice are consistent with malignant peripheral nerve sheath tumors and serve as a useful platform for preclinical experimentation.
17.5 Undifferentiated Pleomorphic Sarcoma
Undifferentiated pleomorphic sarcoma (UPS), previously known as malignant fibrous histiocytoma (MFH), is a common soft tissue sarcoma with a high incidence of subsequent lung metastasis. No specific cell line or genetic mutation has been identified as the defining characteristic of UPS, and it is not known whether these tumors have a common cell line or if they represent a more diverse group of tumors arising from various mesenchymal cells [41]. As such, an animal model that adequately recapitulates this disease has been difficult to generate. Given the known role of both the tumor suppressor gene p53 and the oncogene Kras in the oncogenesis of various sarcomas, a mouse model was made with conditional mutations in both of these genes.
This model is based on the site-specific recombinase Cre, which removes DNA bracketed by the loxP sequence. Transcription of a gene can be initiated by removing a stop sequence before the gene or the entire gene itself can be removed. In this instance, mice heterozygous for wild-type and mutant Kras (G12D) are used [45]. A loxP-stop-loxP sequence which normally prevents the transcription of the mutant Kras is removed by Cre, thus allowing the transcription of the oncogene. These mice are also homozygous for loxP-p53-loxP. With administration of Cre, both p53 genes are removed. In this model Cre is delivered by injecting an adenovirus bearing Cre (Ad-Cre), such that the final result is that Kras is activated, while p53 is suppressed.
The Kras/p53 model allows tumors to develop at specific anatomic sites in a defined amount of time as tumors develop in 2–3 months at the site of the Ad-Cre injection. Additionally, these tumors resemble human UPS in that there is an incidence of lung metastasis in approximately 50 % of mice. Compared to other animal models, tumors which arise most closely mimic human tumors and possess aggressive spontaneous metastatic potential.
17.6 Clinical Applications of Immunotherapy for Sarcoma
To take advantage of unique tumor antigens and the animal models described previously, a basic understanding of tumor immunology is necessary. To determine self from nonself, nucleated cells display type I major histocompatibility complexes (MHC I) and present a repertoire of cellular proteins. Immune surveillance by CD8+ T cells can detect nonself proteins in the context of MHC I by interactions with unique T-cell antigen receptors (TCRs). TCRs confer specificity for a particular target antigen and allow clonal expansion of CD8+ T cells [46]. Activated CD8+ T cells differentiate into cytotoxic T-cell lymphocytes (CTL). Target cell destruction is then induced through the release of inflammatory cytokines such as tumor necrosis factor (TNF) and interferon gamma (IFNγ), the induction of TNF-related apoptosis-inducing ligand (TRAIL), and cytotoxic degranulation which leads to perforin-mediated lysis as an adaptive immunity to intracellular infections [47]. By the expression of specific co-stimulatory molecules, antigen-presenting cells (APCs), such as DCs, are capable of stimulating naive T cells to proliferate and differentiate in response to antigens and are powerful tools for manipulating the immune system [48]. As clinical evidence of the potency of DCs to influence immune responses, a set of ten pediatric patients with solid tumors underwent a DC vaccination protocol [49]. DCs were pulsed with tumor cell lysates and the immunogenic protein keyhole limpet hemocyanin, which generated significant regression of multiple metastatic sites in one patient and stable disease in five patients.
Unfortunately, tumor cells often lose MHC class I molecules from their cell surface, thereby escaping recognition by CD8+ T cells [50–52]. While loss or downregulation of MHC I should lead to poorer clinical outcomes, results are mixed. When prognostic significance of MHC I expression was analyzed, patients with osteosarcoma and high expression of MHC class I showed significantly better overall and event-free survival, but no prognostic significance was found in patients with malignant fibrous histiocytoma [53]. In contrast, in patients with Ewing sarcoma family of tumors, downregulation or negative expression of MHC class I was associated with poorer survival [54].
17.6.1 Adoptive Cell Therapy
Adoptive cell therapy (ACT) harnesses a cancer patient’s own antitumor T lymphocytes, which are expanded ex vivo into large numbers. These expanded cells are then reinfused back into the patient to achieve an antitumor effect. ACT allows several opportunities for immune modulations that can feasibly be applied to any tumor, including sarcoma, but have been most successfully applied to melanoma. Immune modulations include the selection of highly active, tumor-reactive lymphocyte cultures with optimal characteristics, rapid expansion of ex vivo lymphocyte cultures which circumvent immune regulatory mechanisms and the potentially suppressive tumor environment, and host systemic immunosuppression prior to cultured lymphocyte infusion to further enhance activity [55]. The discovery that normal human lymphocytes can be genetically engineered to recognize cancer antigens and mediate cancer regression in vivo has opened opportunities for improving and extending the ACT approach to patients with a wide variety of cancer types [56]. The potential success of this approach for sarcomas has been evident for decades. In an early study, tumor-infiltrating lymphocytes (TILs) from transplantable mouse sarcomas were cultured in high levels of IL-2 where they showed specific lytic activity toward the cognate tumor cells in vitro and also mediated tumor regression when transferred into tumor-bearing, cyclophosphamide-conditioned mice [57].
17.6.1.1 Lymphokine-Activated Killers (LAKs)
In 1982, Grimm et al. demonstrated that IL-2 could activate peripheral blood lymphocytes to generate lymphokine-activated killer (LAK) cells capable of lysing human tumor cell lines [58]. The culture of peripheral blood lymphocytes (PBLs) with IL-2 generates NK cells, nonspecific T cells, and LAKs. While there has been moderate success with decreased size and number of pulmonary sarcoma metastases in a murine model, the limited ex vivo expansion and the cytolytic activity in vivo represent significant barriers [59–61]. The first clinical study combining LAK cells and IL-2 was initiated by Rosenberg and colleagues in 25 patients with advanced cancer [62]. By the end of the 1990s, all published phase II and phase III randomized trials using LAK therapy in cancer showed a clinical response rate of about 15–20 %, not superior to IL-2 monotherapy or IL-2 combined with IFN-α [63, 64].
17.6.1.2 Cytokine-Induced Killers (CIKs)
Cytokine-induced killer (CIK) cells are a heterogeneous population of effector CD8+ T cells with diverse TCR specificities, possessing non-MHC-restricted cytolytic activities against tumor cells [65]. CIKs are generated by the addition of IFNγ and IL-1α to IL-2 in culture. Human CIK cells have been shown to have enhanced cytotoxicity and to proliferate more rapidly than lymphokine-activated killer (LAK) cells by both in vitro and in vivo studies. CIKs have been characterized as having a higher lytic activity when compared to LAKs, mainly due to the higher proliferation of CD3+CD56+ cells and to the increased cytotoxic activity of TCR-alpha/beta+ cells in CIK cell cultures [66].
17.6.1.3 Natural Killers (NKs)
Natural killer (NK) cells are lymphocytes important to the innate immune responses, especially against bacteria and viruses. Unlike T or B cells, they do not require clonal expansion and differentiation and use cytokines to induce apoptosis. NKs are typically stimulated by distressed cells by the release of IL-12, IL-15, IL-18, and type I interferons, while MHC type I is often responsible for inhibition of NKs. To allow inhibition and activation of NKs, surface receptors such as Killer-cell immunoglobulin-like receptor (KIR) are used, as seen when MHC-expressing cells suppress NK [67, 68]. The loss of MHC antigen expression in a mouse lymphoma and the loss of MHC in human tumors were first recognized in the 1970s, where 25–75 % of tumor cells had MHC downregulation or losses and normal cells remained expressive [69]. Based on their “missing-self hypothesis,” Ljunggren and Kärre hypothesized that NKs could target and lyse lymphoma cells due to the absence of or reduced MHC expression [70]. Therefore, it is conceivable that NK cells function not only as part of the innate immune system but also in adaptive immune responses against tumors that lack MHC expression.
In the development of a novel pediatric therapy, NKs collected from peripheral blood of healthy adult patients were sensitized with Ewing sarcoma, rhabdomyosarcoma, neuroblastoma, and osteosarcoma cell lines [71]. After in vitro studies, Ewing sarcoma and rhabdomyosarcoma cells appeared to be sensitive to the cytotoxicity of expanded and activated NK cells. Interestingly, as another example of NK cell activity in human sarcomas, it was observed that the tyrosine kinase inhibitor imatinib showed clinical benefit in gastrointestinal stromal tumors (GISTs) that lacked the mutant isoforms of KIT and PDGRF receptors. It was found that imatinib acted on DCs to promote NK cell activation in vitro and in vivo, where NKs generated an enhanced tumor response in vivo [72].
17.6.1.4 Engineered T Cells
Another approach to ACT involves genetically engineered T cells to express a specific tumor antigen-recognizing TCR. While several studies showed that transfer of TCR-engineered peripheral blood lymphocytes allowed recognition of tumor cells, these studies were mainly for the treatment of melanoma [73–75]. However, one unique study focused on sarcoma. NY-ESO-1, a cancer-testis antigen previously discussed, is expressed in 80 % of synovial cell sarcomas [76]. Using autologous T cells transduced against metastatic synovial cell sarcoma expressing NY-ESO-1, an objective clinical response was seen in four of six patients and a partial response lasting 18 months in one patient following adoptive transfer [77].
17.6.1.5 Chimeric Antigen Receptors (CARs)
The production of T cells expressing chimeric antigen receptors (CARs) is a novel treatment for osteosarcoma [78]. CARs were generated to enable T cells to overcome mechanisms by which tumors escape from immune surveillance including tumor cell MHC I downregulation or loss [79]. The structure of the CAR is comprised of an exodomain, typically derived from the antigen-binding portion of a monoclonal antibody linking the VH and VL domains to construct a single-chain fragment variable region. By doing so, CARs are highly targeted and allow antigen recognition even in tumors with MHC loss [78]. Using human epidermal growth factor receptor 2 (HER2)-specific CARs to overcome low expression of HER2 in a locoregional and metastatic mouse model, these genetically modified T cells demonstrated the ability to mediate regression of osteosarcoma tumors [80].
17.6.2 Sarcoma Immunotherapy of the Future: CTLA-4 and PD-1 Manipulation
17.6.2.1 CTLA-4
Found on the surface of activated and regulatory T cells and located on chromosome 2q33 is cytotoxic T-cell lymphocyte antigen-4 (CTLA-4), also known as cluster of differentiation 152 (CD152). While it is known that CD28 is an activator of T cells, CTLA-4 is a known inhibitory receptor. Both CTLA-4 and CD28 bind to CD80 and CD86, also called B7-1 and B7-2 respectively, on antigen-presenting cells. Early preclinical testing in colon carcinoma as well as fibrosarcoma suggested that blockade of CTLA-4 can lead to tumor cell recognition and elimination [81]. This led to successful clinical testing for metastatic melanoma where clinical responses were seen in 13–21 % of patients [82, 83]. Unfortunately, testing of anti-CTLA-4 monoclonal antibodies for various other cancers has yet to be as successful. In an in vivo mouse lymphoma model, CTLA-4 blockade enhanced the priming of T cells from vaccination, but did nothing to prevent the tolerance to tumor cells [84]. Similarly, a recent phase II trial of a CTLA-4-blocking antibody in patients with synovial sarcoma showed no clinical responses, nor was there a clinical benefit of antitumor antigen serological responses [85]. While data are mixed regarding the use of CTLA-4 blockade and antitumor activity, it is possible that blockade alone may not be adequate for continued tumor control.
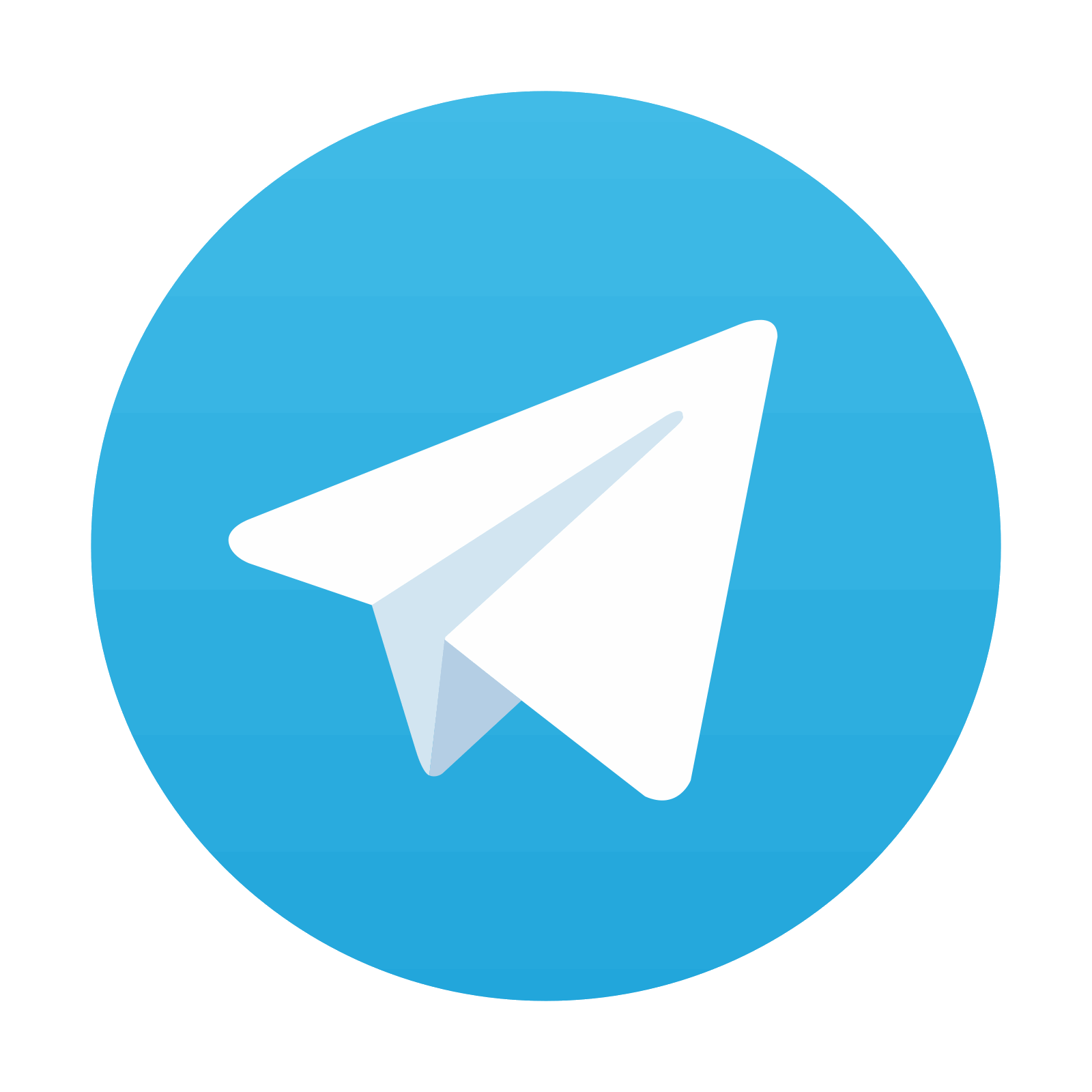
Stay updated, free articles. Join our Telegram channel
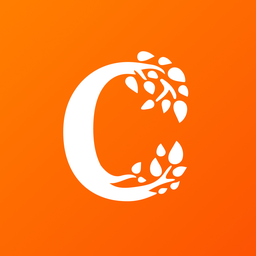
Full access? Get Clinical Tree
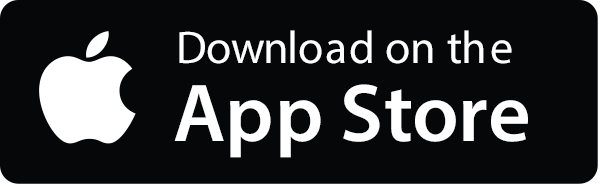
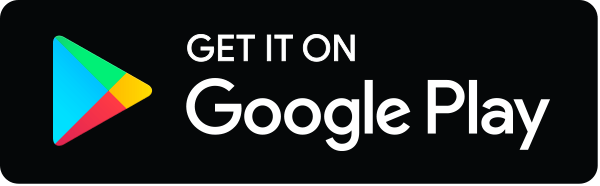