Fig. 5.1
Models of pathogenesis of AML. Three possible scenarios for the development of the LSC are shown. Hematopoietic stem cells (HSC) or myeloid progenitors (MP) or both populations are potential targets for primary (Hit I) and secondary (Hit II) hits. Usually, one single mutation leads to a preleukemic stem cell (pre-LSC) of myeloid progenitor (Pre-LMP), and a second mutation (Hit II) results in the formation of a leukemic stem cell (LSC) that finally gives rise to the bulk of the leukemic blasts, although the LSC can also – albeit to a lower extent – lead to normal differentiated cells. The role of epigenetic changes (Hit III) has recently become clear, but the exact mechanism has not been fully unraveled
The role of miRNAs in the development of AML, as in other cancers, has been shown by several studies comparing miRNA signature between AML and ALL, between AML and normal CD34+ cells, and different AML samples (reviewed in [35]).
5.2.4 The Leukemic Stem Cell
5.2.4.1 Phenotype of the LSC
Most AML cells are unable to proliferate extensively, and only a subset of these cells preserves clonogenic properties, suggesting that, similar to normal hematopoiesis, leukemia may be maintained by a small population of stem cells [15–17, 36, 37]. In 1994, Dick and colleagues identified that AML-initiating cells or LSCs are CD34+CD38− (a phenotype that is similar to normal HSC), based on transplantation experiments in SCID and later NOD/SCID mice [15, 16]. In addition, serial transplantation experiments performed by Shultz et al. in xenotransplant-permissive NOD/SCID/IL2Rγ–/– (NSG) mice demonstrated that long-term engraftment and the self-renewal capacity of human AML cells resided exclusively in the CD34+CD38− population [38]. LSCs were shown to be mainly in the G0 phase of the cell cycle, confirming their quiescent nature [38, 39].
Despite these studies, controversy about the immunophenotype of the LSC arose (Table 5.1) [40]: it is now clear that although the LSC is contained within the CD34+CD38− population in most patients, some exceptions exist where LSC can (also) be found in the CD34+CD38+ population [15, 16, 21] or the CD34low/– fraction (K, I) [21]. Moreover, identifying a more refined immunophenotype discriminating LSC from normal HSC would enable clinicians to better evaluate MRD after therapy and design LSC-targeted therapies. Some surface markers associated with LSC are C-type lectin-like 1(CLL-1/MICL/CLEC12A), CD123, CD44, CD47, CD96, and CD25 [41–47], but still a unique phenotype has not been established thus far.
Antigen | Examples | Advantages | Disadvantage(s) |
---|---|---|---|
LSA | > mutations: Flt3, NPM1 | Specificity | Expression restricted to defined AML subgroups → use limited to small patient populations |
> translocations: AML1-ETO, DEK-CAN, PML-RARα | Oncogenicity | ||
LSC expression | |||
LAA | WT1, AurAkinase, Bcl2, Muc1, SSX21P | LSC expression | Low avidity of T cells for AG |
Broad applicability (AML and other types of cancer) | Potential toxicity to normal tissues | ||
MIHA | HA-1, HA-2 (hematopoietic specific) | High-avidity T cells available | Use limited to allo-HSCT |
AG recognized by both CD4+ and CD8+ T cells | Limited number of AG defined | ||
Potential multivalent response | Potential cause of GVHD |
5.2.4.2 Clinical Relevance of the LSC
If LSC, as defined in mouse models, were also relevant for AML patients, they may constitute the main targets for consolidation therapy against MRD [48]. In 2005, Van Rhenen et al. demonstrated that a high frequency of CD34+CD38− LSCs at AML diagnosis predicts high frequencies of MRD after chemotherapy and poor overall, disease-free and relapse-free survival, both in an in vivo model and in correlation studies in patients [49]. Another study reported that the relative ability of AML cells to successfully engraft in immunodeficient mice (a property associated with LSCs) correlates with adverse clinical features [50]. Recently, two groups have independently demonstrated that HSC- and LSC-enriched populations share very similar transcriptional “stem cell-like” or “self-renewal” gene expression signatures that reflect stem cell function in vivo [51] and that are predictive of adverse clinical outcome in individuals with AML [51, 52]. The predictive value of this LSC score appeared to be independent of other risk factors in multivariate Cox regression analysis, which further supports the clinical relevance of LSC [51, 52].
5.2.5 How Do Gene Alterations in the LSC Lead to the Clinical Presentation of AML?
Genetic alterations lead to differentiation block and hyperproliferation, which further enhance the risk of genetic damage. This leads to a number of effects that are additive and ultimately lead to the clinical effects of this life-threatening disease:
Clonal outgrowth and uncontrolled, limitless expansion, which is achieved by mutations that lead to constitutive activation of pathways involved in cell cycle, e.g., by activating mutations, overexpression of proto-oncogenes, and abrogation on restriction points [53].
Inefficient maturation from the malignant blasts and also mature cells from the residual normal stem cells, caused by a maturation arrest (due to mutation), by cytokines that are produced by the malignant blasts and inhibit the normal differentiation, and – to a lesser extent – by the crowding effect
Constitutive release of chemokines by the malignant blasts (that also express several chemokine receptors), which interact with other cytokines (esp hematopoietic growth factors and angioregulatory factors), and matrix metalloproteinases (MMP) system, also released by the AML cells [54]
Expression of P-glycoprotein (Pgp, MDR1, ABCB1), plasma membrane transporters able to efflux a variety of substrates from the cytoplasm, including chemotherapeutic agents, leading to the development of resistance to chemotherapy [55].
Resistance to apoptosis and defective or proficient DNA damage response [56]
5.3 Immunotherapy for AML
Despite the progress that has been made in the past decades, AML still remains a therapeutic challenge. A significant percentage of patients, especially the elderly, have primary induction failure, and even if chemotherapy is successful at inducing remission of AML, the probability of relapse is high [56]. Immunotherapy for AML was first put forward almost 40 years ago: the hypothesis that AML blasts were distinct from normal blasts led to preliminary attempts to improve immune responsiveness to AML by the administration of inactivated autologous AML blasts with BCG [57]. The most important insights in the role of the immune system in controlling AML, however, came from allogeneic HSCT and the observed graft-versus-leukemia (GVL) effect. Increasing evidence exists that the success of allo-HSCT in curing AML can be largely attributed to this GVL effect, especially in the context of non-myeloablative HSCT [58–62]. Both donor NK cells and donor T cells contribute to the suppression and elimination of leukemic cells [62]. Although allo-HSCT remains the most successful post-remission therapy in AML, it has its price of important morbidity and mortality, caused by infections, toxicity of the conditioning, and acute and chronic graft-versus-host disease (GVHD) [58, 59]. These important complications limit its applicability in patients of older age and with comorbidities. Therefore, research groups investigated more targeted forms of immunotherapy that are more specific, do not require conditioning, and have less side effects. The biggest challenge here lies in identifying the ideal tumor antigen, present on LSCs, but low to absent on normal hematopoietic cells and other vital tissues.
5.3.1 Antigens to Target in AML
5.3.1.1 Antigens Presented by MHC After Internal Processing
Major histocompatibility complex (MHC)-presented antigens are targets for T cell-mediated immunotherapy, such as vaccination and adoptive T cell therapy. Three types of MHC-presented antigens can be described in AML: leukemia-specific antigens (LSA), leukemia-associated antigens (LAA), and minor histocompatibility antigens (MIHA). LSA arise from mutations or translocations, which lead to the formation of new antigens specific for the AML cells. LAA are expressed both on normal and leukemic cells, but they can be good targets if they are overexpressed on leukemic cells (including LSC) and/or their physiological expression is restricted to certain developmental stages (embryologic) [62–65]. Hematopoietic-specific MIHA differing between donor and recipient are interesting targets for AML in the context of allo-HSCT [61, 62, 66–70]. A ranking of the most promising cancer antigens is reviewed by Cheever et al. [67]. In addition, the authors and other groups have published an overview of AML antigens more recently [40, 71, 72]. Some examples, the advantages and disadvantages of these antigen types are summarized in Table 5.1.
5.3.1.2 Surface Antigens
Surface antigens are targets for monoclonal antibodies (mAbs) and chimeric antigen receptor-modified T cells (CAR T cells). Surface antigens on AML that are being targeted by mAbs are CD33, Flt3L, and those overexpressed on LSCs (CLL1, CD44, CD47, IL-3R (CD123)) (reviewed in [73]). These antigens have the disadvantage of being also expressed on normal tissues, resulting in important side effects, as seen with the anti-CD33 antibodies [73, 74] (See Sect. 5.3.2.2.1).
5.3.2 Current Immunotherapeutic Strategies for AML
Active immunotherapy (e.g., modified leukemic cells, peptide, DNA, or dendritic cell-based vaccinations) requires a patient with an intact immune system and can only exploit the available T cell receptor (TCR) of the patient. However, high-affinity TCR-bearing T cells specific to self-antigens (TAA) are expected to be deleted after negative selection in the thymus. In addition, the question is whether active immunotherapy will be able to combat the abundant negative influences of the host immune system and tumor microenvironment. Passive immunotherapeutic strategies (e.g., adoptive transfer of AML-specific T cells or NK cells) are expected to be more potent therapies to target LAA and MIHA. Also mAbs are considered passive immunotherapy and have proven efficacy in AML.
5.3.2.1 Active Immunotherapeutic Strategies
Peptide Vaccination
Known immunodominant and HLA-A2 (being highly prevalent among Caucasians)-binding nonamer peptide epitopes of WT1 and proteinase 3 (PR1) are most widely researched and developed as peptide vaccines for AML in clinical trials. Receptor for hyaluronan-mediated motility (RHAMM) has also been targeted in vaccine trials [75–77]. Vaccines have been combined with adjuvants such as montanide or keyhole limpet hemocyanin (KLH), with or without concurrently administered granulocyte-macrophage colony-stimulating factor (GM-CSF). As these studies comprise small and diverse groups of patients treated with different vaccines and schedules, it is difficult to draw meaningful conclusions about the true efficacy of peptide vaccination in AML. Yet, immune responses were significantly associated with clinical response. Clinical responses ranged from reduction in marrow blasts to complete remissions in a low percentage of patients The potency of peptide vaccines may potentially be increased by genetically modifying peptides to enhance TCR affinity or by the use of synthetic long peptides (SLP) (instead of exact MHC-binding nonamer peptides), which deliver antigens in a more efficient and stable way to the patient’s antigen presenting cells (APCs) [78, 79]. In addition, Toll-like receptor (TLR) ligand-peptide conjugates constitute an attractive vaccination modality, sharing the peptide antigen and a defined adjuvant in one single molecule [79, 80].
Dendritic Cell Vaccination
In order to circumvent the limitations inherent to peptide vaccines [81], researchers have intensely studied the role of antigen-loaded dendritic cells (DCs) as professional APCs which are able to prime naïve T cells [82]. Furthermore, the synthetic peptide approach which may miss immunodominant epitopes is replaced by the addition of whole protein or mRNA transfection [81]. Most of the strategies use DCs which are derived from monocytes, and only those strategies used in clinical trials are mentioned here:
DCs pulsed with leukemic cell lysates [83], apoptotic leukemic cells [84], or modified WT1 peptides [84] have been successfully explored in small clinical trials.
Even more promising are mRNA-electroporated DCs. In 2010, a phase I/II clinical trial (clinicaltrials.gov ID: NCT00834002) investigated the effect of vaccination with full-length WT1 mRNA-electroporated autologous dendritic cells in ten patients with AML, and in five of them, a molecular remission was reached, although not always persisting [85].
In an attempt to generate WT1-presenting DCs with a longer in vivo persistence, Stripecke and colleagues recently developed a tricistronic lentiviral vector co-expressing a truncated form of WT1, granulocyte-macrophage colony-stimulating factor (GM-CSF), and interleukin-4 (IL-4), which was used for the transduction of human monocytes, leading to very rapid self-differentiation of these cells into “SmartDC/tWT1” that showed very promising potential for the use as immunotherapy against WT1-expressing tumors [86].
5.3.2.2 Passive Immunotherapeutic Strategies
Monoclonal Antibodies
Monoclonal antibodies given their antigen specificity and minimal toxicity may be an excellent AML- and even LSC-targeting therapy. This immunotherapeutic strategy functions through several mechanisms: Antibody-dependent cell-mediated cytotoxicity (ADCC), complement activation, a direct proapoptotic effect, and upon the inhibition of signal transduction cascades that are essential for homeostasis, proliferation, or interaction with the microenvironment [87]. In case mAbs are conjugated to radioisotopes or toxins, they can directly kill the recognized target. Anti-CD33 (present on 90 % of AML cells) mAbs are the most widely studied and have proven both clinical efficacy and important toxicity [72, 74, 88]. Current promising trials combine anti-CD33 mAbs in more fractionated (less toxic) administration with chemotherapy [89, 90]. Also radioisotope-coupled anti-CD45 antibodies have been used as part of the conditioning before allo-HSCT [91]. Alternative mAb tools include antibodies that block the immune-regulatory effect of molecules, such as cytotoxic T lymphocyte antigen-4 (CTL4) or programmed cell death-1 (PD-1), and thereby unleash cytotoxic T lymphocyte function [92–97].
Adoptive T Cell Transfer
The clinical results obtained with unmanipulated DLIs, a variety of T cell types/sources and ex vivo manipulations of T cells, point to a strong AML-directed therapeutic effect as well as a GVHD potential. Various research has been done to direct T cells more specifically toward the AML cells. In AML patients, autologous- or donor-derived antigen-specific T cells can be isolated from peripheral blood by pMHC-multimer staining, CD137- or CD154-based assays, cytokine (IFNγ)-secretion techniques, or repeated ex vivo stimulation with antigen and subsequent expansion, but all of these techniques still require the availability of pre-existing high-affinity antigen-specific T cells in the patient or the donor (in the context of allo-HSCT) [98–100]. For LAA, these are usually absent due to negative selection in the thymus. In order to confine LAA-specificity to T cells, peripheral blood mononuclear cells (PBMC) can be transduced with a high-affinity TCR recognizing LAAs present on AML blasts, including LSCs, thereby circumventing the issue of tolerance. Such a high-affinity TCR can be isolated from LAA-specific T cells generated in vivo or in vitro in an autologous setting (e.g., from TILs of a patient with complete clinical responses after ACT) or, even more ideally, in an allogeneic MHC-mismatched setting [101–103]. Moreover, the availability of TCR genes specific for MIHA, such as HA-2, would increase the applicability of MIHA-directed immunotherapy, illustrated by the fact that 95 % of the population expresses the antigenic HA-2v allele, and therefore, naturally mismatched recipient-donor pairs are infrequent [104].
A new promising immunotherapeutic strategy, using PBMC transduced with a CAR, a construct that encodes the VH and VL domain of a tumor antigen-specific antibody coupled to the CD3ζ chain (alone or combined with the signaling motifs of CD28 or CD137 to enhance the signal) of a TCR [105], has not yet been evaluated in clinical trials of AML.
Adoptive NK Cell Transfer
NK cells have an important antileukemic effect, and their role in other immunotherapeutic strategies has been established, e.g., in WT1-DC vaccination [85, 87] and KIR-mismatched haploidentical HSCT [106, 107]. Especially in the context of haploidentical HSCT, NK cell adoptive therapy is currently being explored in clinical trials (NCT 00799799, NTR 2818).
5.4 Concluding Remarks
This chapter reviewed both the immunopathology of AML and currently explored immunotherapeutic strategies targeting AML. The genetic alterations leading to the differentiation block and hyperproliferation which result in AML were discussed. The potential clinical relevance of the LSC concept in the pathogenesis of AML was emphasized. LSC might be one of the reasons why AML still remains a tremendous therapeutic challenge nowadays. New therapeutic strategies, including immunotherapy, are awaited. Various immunotherapeutic strategies and the possible target antigens were listed.
References
2.
Swerdllow S, Campo E, Harris NL. WHO classification of tumours of haematopoietic and lymphoid tissues. Lyon: IARC Press; 2008.
3.
Purtilo D, Paquin L, Gindhart T. Genetics of neoplasia–impact of ecogenetics on oncogenesis. A Rev Am J Pathol. 1978;91(3):609.
4.
Kralovics R. Genetic complexity of myeloproliferative neoplasms. Leukemia. 2008;22(10):1841–8.PubMed
5.
El Abed RBV, Huiart L, et al. Molecular study of CEBPA in familial hematological malignancies. Fam Cancer. 2009;8(4):581–4.PubMed
6.
Hasle H. Pattern of malignant disorders in individuals with Down’s syndrome. Lancet Oncol. 2001;2(7):429–36.PubMed
7.
Mathew C. Fanconi anaemia genes and susceptibility to cancer. Oncogene. 2006;25(43):5875–84.PubMed
8.
Graubert TA, Shen D, Ding L, Okeyo-Owuor T, Lunn CL, Shao J, et al. Recurrent mutations in the U2AF1 splicing factor in myelodysplastic syndromes. Nat Genet. 2012;44(1):53–7.
9.
Hijiya N, Ness KK, Ribeiro RC, Hudson MM. Acute leukemia as a secondary malignancy in children and adolescents: current findings and issues. Cancer. 2009;115(1):23–35.PubMedCentralPubMed
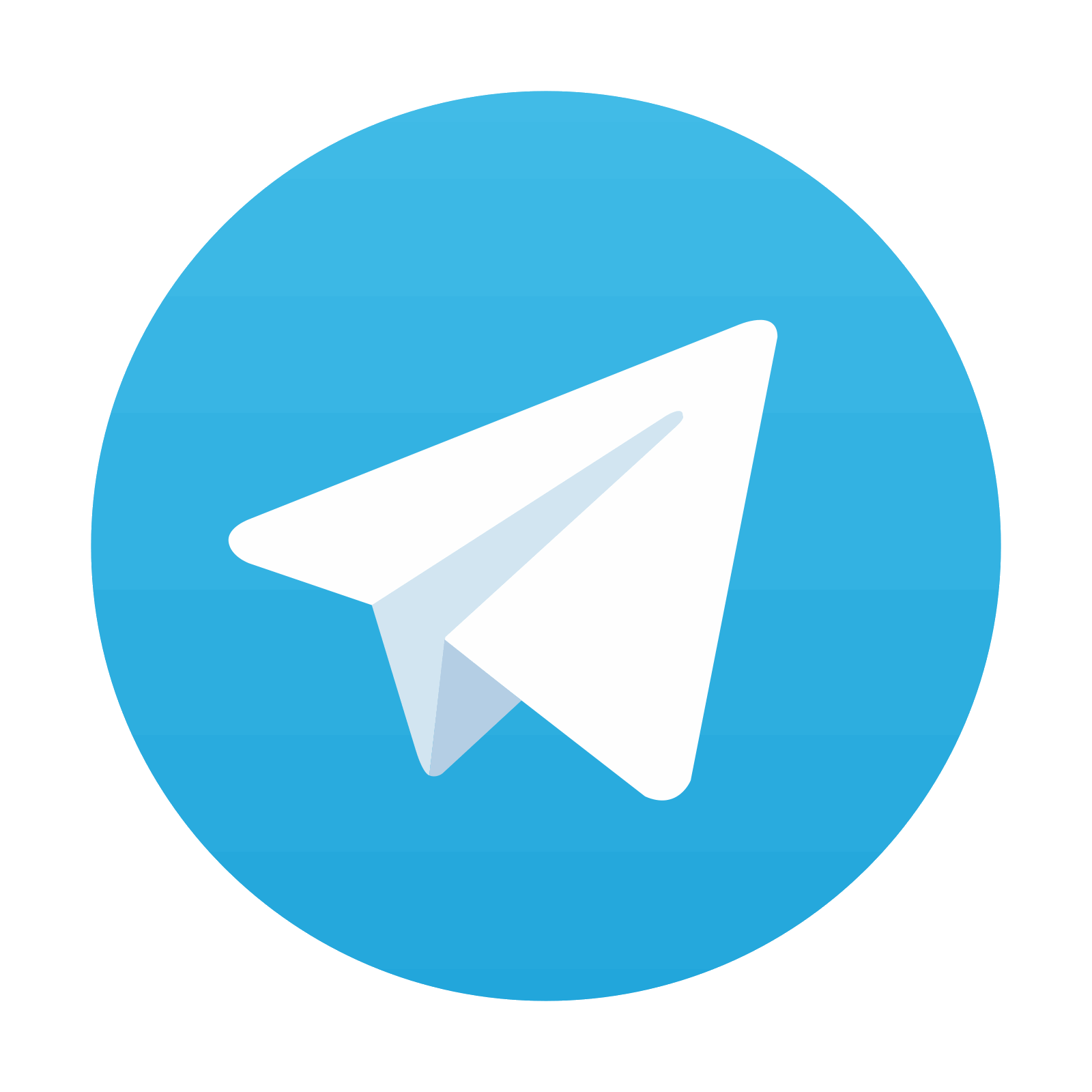
Stay updated, free articles. Join our Telegram channel
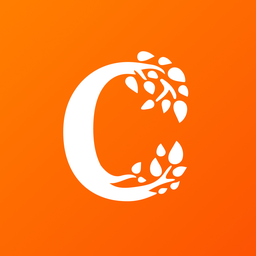
Full access? Get Clinical Tree
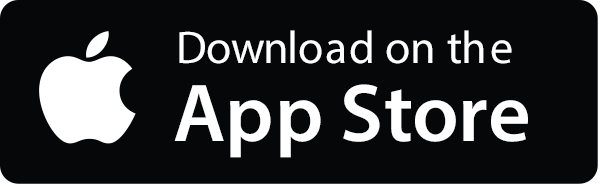
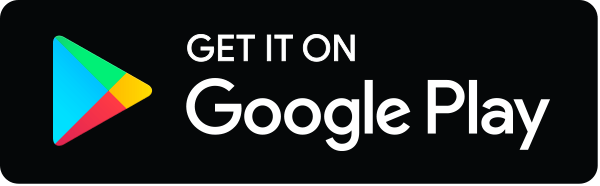