Other Blood Group Systems
As Table 3.1 shows, the Lutheran (Lu), Kell (K), Duffy (Fy) and Kidd (Jk) systems were discovered by finding alloantibodies in the serum of transfused patients or of mothers of infants with haemolytic disease of the newborn (HDN). Other systems since discovered in the same way are Diego (Di), Cartwright (Yt), Xg, Scianna (Sc), Dombrock (Do), Colton (Co), Chido/Rodgers (Ch/Rg), Kx, Gerbich (Ge), Cromer (Cr), Knops (Kn) and Indian (In).
Table 3.1 The first 10 blood group systems to be discovered.
Terminology of Blood Groups
Blood group terminology is inconsistent. In some systems, for example ABO, antigens are given capital letters (e.g. B) or capital letters with subscripts (e.g. A2); the corresponding alleles are denoted by italics, with superscripts when relevant (e.g. A2). Although the alleles of the ABO system are all thought to be alternative forms at a single locus, this is not indicated by the nomenclature (e.g. one allele of A2 is O). In other systems (e.g. Kell) the main alleles are denoted by large and small letters, thus K and k; here the terminology indicates that the alleles are alternative forms. The MNS system follows a blend of these two systems of nomenclature, the first antigens to be described being called M and N, and the next pair S and s.
The Le, Lu, Fy, Jk, Di, Yt, Do, Co, Kn and In systems follow a uniform pattern; the first alleles to be recognized are denoted thus Lua, Lub; phenotypes are written, for example, Lu(a+b−) and the corresponding antibody, anti-Lua. However, in almost all the systems, more than two alleles have been discovered and these additional alleles have usually been given numbers, for example, Fy3. In the Xg, Pl, Hh and Kx systems only one antigen has been demonstrated so far, i.e. Xga, Pl, H and Kx respectively. The silent allele of Xga is termed Xg and that of H is h; those of P1 and Kx have no names. In the Sc system, the antigens are termed Sc1 and Sc2. In most cases the full name of the system is that of the subject in whom the antibody that led to the recognition of the system was first found. For the Rh system there were for a long time two nomenclatures, although now the CDE nomenclature is almost always used in preference to Wiener’s. In several systems (Rh, K) a numerical system has for long been preferred by some for the identification of antigens and antibodies.
A standard numerical nomenclature, consisting of six numbers was devised by a Working Party of the International Society of Blood Transfusion (Lewis et al. 1985) and is regularly revised (Daniels et al. 2003). The first three numbers represent the blood group system, for example 001, ABO; 002, MNSs; 003, P; 004, Rh, and the second three numbers represent a particular antigenic specificity within that system, for example 001001, A; 004001, D.
In total, 33 blood group systems have been given numbers so far. In numerical order, systems 0001–033 are: ABO, MNS, P1Pk, RH, LU, KEL, LE, FY, JK, DI, YT, XG, SC, DO, CO, LW, CH/RG, H, XK, GE, CR, KN, IN, OK, RAPH, JMH, I, GLOB, GIL, RHAG, FORS, JR and LAN. In the standard nomenclature, designed to be suitable for computers, the initials of the system are given in capitals, for example RH, but we have preferred to keep to the old style (Rh) in this book.
Further series of numbers have been established to encompass related families of antigens (‘collections’) as well as those high-frequency antigens (‘public’ antigens, the 901 series) and those low-frequency antigens (‘private’ antigens, the 700 series), which are not currently known to be genetically linked to any of the established 33 systems.
The Relative Clinical Importance of Different Blood Group Systems
The clinical importance of a blood group system depends mainly on (1) the frequency with which alloantibodies of the system occur and (2) the characteristics of the alloantibodies, namely, Ig class and, if IgG, subclass, thermal range and ability to fix complement. These characteristics in turn determine the capacity of the antibody to cause red cell destruction and to be transferred across the placenta and cause HDN.
In the ABO and Lewis systems, the presence of soluble blood group antigens in the plasma is sometimes of clinical significance. In the fetus and newborn infant, A and B in the plasma may be partially protective against maternal anti-A and -B; and in transfusion recipients with potent anti-Lea, who are transfused with Le(a+) blood, the presence of Lea in the donor’s plasma neutralizes the antibody and protects against red cell destruction.
ABO is easily the most important blood group system because anti-A and -B are found as naturally occurring antibodies in virtually all subjects who lack the corresponding antigens and these antibodies often cause intravascular haemolysis when incompatible red cells are transfused. The Rh system is next in importance because Rh D-negative subjects readily form anti-D and this antibody is capable of causing haemolytic transfusion reactions and HDN.
There are some systems in addition to ABO in which naturally occurring antibodies are sometimes found but these antibodies are usually inactive at body temperature and are then incapable of causing red cell destruction. Naturally occurring antibodies active at 37°C are often found in the Lewis system but, for reasons described later, very seldom cause haemolytic transfusion reactions.
There are other systems that have some similarities to Rh in that naturally occurring antibodies are rare and immune antibodies relatively frequent, particularly in patients who have been transfused many times. The most important of these systems, Kell, is very much less important than Rh, and the importance of the many other blood group systems, also described in Chapter 6, becomes progressively less until one reaches a system such as Colton in which very few antibodies have ever been encountered.
Red Cell Antigens
The Red Cell Membrane
Like other cell membranes, the red cell membrane (see Colour Plate 3.1) is an assembly of lipid and protein molecules. The lipids, which together make up almost one-half of the mass of the membrane, are phospholipids (60%), cholesterol (30%) and glycolipids (10%). The lipid molecules are arranged as a continuous double layer, 4–5 nm thick.
The properties of mechanical strength and deformability that the red cell exhibits allow it to undergo the extensive deformation in the microvasculature necessary for O2 delivery to the tissues over its 120-day lifespan. These properties are imparted by the skeleton, a network of proteins lying just beneath the red cell membrane, and tethered to the lipid bilayer through interactions involving the transmembrane proteins band 3 and glycophorin C. Tetrameric band 3 is part of a macrocomplex of proteins which includes the Rh (D and CE) proteins, RhAG, GPA and GPB and the minor red cell surface proteins CD47 and LW glycoprotein (ICAM-4). Interaction of this band 3 complex with the underlying skeleton is mediated by interactions with ankyrin (Colour Plate 3.1). Glycophorin C is located in another protein complex and here the interaction with the skeleton is mediated through band 4.1, actin and adducin. The glycophorin C complex is thought to contain dimeric band 3 and Rh proteins as well as the minor red cell surface proteins Kell, Kx and Duffy. An interaction between dimeric band 3 and adducin is important for maintaining membrane stability (Colour Plate 3.1; Salamao et al. 2008; Anong et al. 2009). Evidence has also been presented that the glucose transporter (GLUT 1) provides another link to the skeleton through interaction with dematin in the glycophorin C complex (Khan et al. 2008; Anong et al. 2009). GLUT 1 through interaction with stomatin regulates a switch from glucose transport to transport of an oxidized form of vitamin C (L-dehydroascorbic acid) in human erythrocytes and the few other mammals that have lost the ability to synthesize ascorbic acid from glucose (Montel-Hagen et al. 2008). The major component of the skeleton, spectrin, consists of two subunits α (240 kDa) and β (220 kDa), which associate to form heterodimers. The heterodimers associate head to head to form tetramers. The tails of the tetramers interact at the glycophorin C complex with a variety of proteins, including actin, protein 4.1, dematin (protein 4.9), tropomyosin and adducin (Gilligan and Bennett 1993; Peters and Lux 1993).
Ankyrin, in association with protein 4.2 (pallidin) attaches spectrin tetramers to the amino terminal cytoplasmic domain of the transmembrane protein band 3.
The term ‘Band’ and the numbers given above, for example 4.1, refer to results obtained by the technique known as SDS-PAGE. In this technique, washed red cell ghosts are mixed with the ionic detergent sodium dodecyl sulphate (SDS), which solubilizes membrane proteins by displacing lipids from transmembrane regions. The solubilized proteins can be separated according to size by electrophoresis through a polyacrylamide gel, which acts as a molecular sieve: large glycoproteins tend to move more slowly than small ones (PAGE = polyacrylamide gel electrophoresis). When the separated proteins were visualized by staining with a protein stain (Coomassie blue) a number of bands were seen and these were numbered according to their apparent molecular size so the band of highest apparent molecular weight became band 1, the next largest band 2 and so on (Steck 1974). In the intervening years some of the bands numbered in this way have acquired other names, the two subunits of spectrin were originally bands 1 and 2, while others, band 3, band 4.1 and band 4.2, have remained in common usage.
Surface Proteins of the Red Cell
The blood group antigens of 23 blood group systems (MNS, Rh, Lu, K, Fy, Jk, Di, Yt, Xg, Sc, Do, Co, LW, Kx, Ge, Cr, Kn, In, Ok, Raph, JMH, GIL and RhAG) are intrinsic red cell surface proteins. The primary sequences of all these proteins are known and in most cases the molecular bases of the antigens in the systems are also known (see Chapters 5 and 6). These blood group active proteins constitute a diverse array of structures and functions. A minority of proteins at the red cell surface are not known to express blood group antigens defined by their protein sequence at the time of writing. These include the glucose transporter (GLUT1), CD47 (IAP), CD58, CD59(MIRL), lactate transporter (MCT-1), prion protein (PRP) and a nucleoside transporter. The major surface proteins (defined as those with an abundance of 200 000 copies/red cell or more), and most of the minor surface proteins (50 000 copies/red cell or less), are listed in Table 3.2, and models of the structures of some of the blood group active proteins are shown in Colour Plate 3.2. Some of the minor proteins (LW, CD47) associate with the major protein band 3 complex depicted in Colour Plate 3.1 while others, particularly the GPI-anchored proteins, are located in microdomains known as ‘lipid rafts’ in areas of the red cell membrane remote from the major skeletal linked protein complexes (Brown and London 2000). The antigens of the Ch/Rg system are defined by the protein sequence of the fourth component of complement (see Chapter 6). The antigens of the remaining six systems (ABO, P, Le, H, I and Globoside) are formed by the action of glycosyltransferases and these are discussed in detail in Chapter 4. Carbohydrate structures at the red cell surface occur on both proteins and lipids and so antigens defined by carbohydrate structure alone are on several different molecules. There are two major oligosaccharide stuctures found on proteins, N-glycans and O-glycans. N-glycans are formed by the attachment of sugars to asparagine (N) in the polypeptide chain when it is part of a signal sequence N-X-S/T, where X is any other amino acid except proline and S/T is serine or threonine. N-glycans can be very large branched structures (Kameh et al. 1998). O-glycans are heavily sialylated and generally smaller than N-glycans. They are found mainly on the glycophorins but other proteins may also carry them (CD55 syn DAF for example, Lukacik et al. 2004).
Table 3.2 Surface proteins of the red cell (based on Anstee 1990, 1993).
The A, B, H, I and i antigens are carbohydrate structures (N-glycans) carried mainly on Band 3 and the glucose transporter (GLUT1). These proteins together account for about 2 million potential N-glycans/red cell but other glycoproteins, for example RhAG and aquaporin 1, and glycolipids also carry these antigens; see the section on the chemistry of ABH and other carbohydrate antigens in Chapter 4.
Transmembrane proteins contain stretches of around 20 hydrophobic amino acids (transmembrane domains), which lock them into the double layer of phospholipids in the cell membrane. There are three structural types of transmembrane protein in the red cell membrane. Many are type I membrane proteins, having a single membrane traverse with the amino terminus on the outside of the cell and the carboxyl terminus on the inside (glycophorins A, B and C, Lutheran, Xg, Sc, LW, Kn, In, Ok, CD47). The Kell protein is a type II membrane protein. It has a single transmembrane domain but its amino terminus is on the inside of the red cell and its carboxyl terminus on the outside. Several transmembrane proteins have more than one transmembrane domain and so are predicted to pass through the lipid bilayer several times, band 3 (14 times), glucose transporter (10 times), aquaporin 1 (six times), Rh polypeptides and Rh glycoprotein (RhAG, 12 times each), Jk protein (urea transporter, 10 times), Kx protein (10 times), Fy protein (DARC, seven times), Raph glycoprotein (CD151, four times), nucleoside transporter (12 times) and GIL protein (aquaporin 3, six times). Other proteins are attached to the membrane through a glycosylphosphatidyl inositol (GPI) anchor and do not have transmembrane domains (Yt (AchE), Cr(CD55,DAF), CD58, Do, JMH(CDw108), CD59(MIRL) and prion protein (PRP) (see Colour Plate 3.2). Notable in this latter group are the complement-inhibiting proteins DAF (decay-accelerating factor, CD55), MIRL (membrane inhibitor of reactive lysis, CD59) and C8bp (C8 binding protein), also known as HRF (homologous restriction factor). Patients with paroxysmal nocturnal haemoglobinuria have deficiencies in the enzymes that assemble the GPI anchor and so lack all GPI-linked proteins from their red cells. Their red cells are highly sensitive to lysis by complement primarily because of the lack of CD59 (Inoue et al. 2003). Further information about red cell antigens carried on proteins is given in Chapters 5 and 6.
The Antigenic Determinant
The antibody-binding portion of an antigenic determinant, the ‘epitope’, which is the region of the antigen that is complementary to the combining site of the antibody, has an area of between one and seven sugar residues (0.5–3.5 nm) for the carbohydrate moiety of glycoproteins or glycolipids. That part of the antigenic determinant that is bound most strongly is called the immunodominant group and is often the terminal non-reducing sugar residue. Antigenic determinants may be sequential, as in linear polysaccharides or polypeptides, or may be conformational and involve structures brought into proximity by folding, as in the Rh blood group proteins (see Chapter 5 and Plate 5.2).
Inheritance of Red Cell Antigens
Two similar sets of chromosomes – one set inherited from each parent – are present in all cells of the body except the germ cells; each set is composed of 22 autosomes and one sex chromosome so that the total number of chromosomes is 46. In the female, the sex chromosomes are equal in size and are termed X chromosomes but in the male there is one X chromosome and one much smaller chromosome, termed Y.
A simple but incomplete definition of a gene is that segment of chromosomal DNA which determines a particular polypeptide. Alternative forms of genes at a particular locus are termed alleles, for example A, B and O. With one exception (see below), for all genes carried on autosomes an individual may be homozygous (both alleles the same, e.g. AA) or heterozygous (alleles dissimilar, e.g. AO). Whereas the term genotype is used for the sum of inherited alleles of a particular gene, for example AA, AO, phenotype refers only to the recognizable product of the alleles. As O has no serologically recognizable product, the genotypes AO and AA both have the phenotype A. The only X-borne blood group genes are Xga and XK, the gene which determines Kx. Females may be homozygous or heterozygous for Xga (XgaXga or XgaXg) but males can only be Xga or Xg and are said to be hemizygous.
Although O has no recognizable product, the DNA structure of this silent allele differs only slightly from that of the A and B alleles. However, in the Rh system, the gene D has no allele. At the corresponding position on the homologous chromosome, there is either another D gene or a D gene altered in such a way that D polypeptide cannot be made. Subjects who have D on either or both of the pair of chromosomes concerned are D positive and those in whom D is lacking from both chromosomes are D negative.
A distinction between the red cells of some homozygotes and heterozygotes (e.g. DD and D, AA and AO) cannot be made reliably with serological methods but can be achieved using molecular methods (see Chapter 12).
Genes that are far apart on the same chromosome, for example Rh and Fy, or genes that are carried on different chromosomes are inherited independently and the antigens they determine are said to belong to different blood group systems; see Table 3.1 for an example and see the following section for the chromosomal location of the different blood group genes.
In bacteria, genes consist of a single stretch of DNA, which directly encodes its protein product but in man (and in all eukaryotes) the coding region is almost always interrupted by at least one stretch of DNA, known as an intron, which does not code for a protein. The introns are spliced out of the RNA prior to its translation into the protein. The coding sections are referred to as exons.
In some cases, more than one protein is produced from a single gene and thus one allele determines more than one polypeptide (see Ge in Chapter 6).
Red cell antigens that are proteins (e.g. Rh) are direct products of genes, but those that are carbohydrate (e.g. A) are determined indirectly by enzymes (glycosyltransferases) that are the gene products; these enzymes transfer the appropriate sugar, determining specificity, on to a structure whose synthesis may be determined by one or more unrelated genes.
In most cases there appears to be a simple correspondence between genes and antigens, so that if a person inherits a given gene the antigen can be detected on the red cells (Figure 3.1). It is not uncommon for one gene to interfere with the expression of another, carried on a different chromosome. For example, the expression of Le is modified by A and B (see Chapter 4) and that of Lu may be modified by mutated erythroid transcription factor genes (GATA-1 and EKLF, see Chapter 6).
Figure 3.1 Independent inheritance of the ABO and Kell genes. In this example, the father passes on A to one son and B to the other, but passes on K to both.
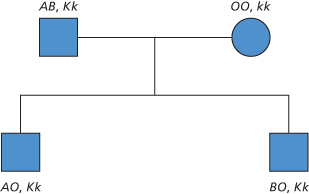
Gene (Allele) Frequencies
Gene frequency means simply the frequency of the allele in the population as a whole; the term allele frequency would be more precise but it is not generally used. From a knowledge of the frequencies of the gene (allele) in a blood group system, phenotype frequencies are readily derived. For example, based on testing almost 200 000 white British subjects, the frequencies of the three main alleles in the ABO system were calculated to be: A, 0.2573; B, 0.0600; and O, 0.6827 (Dobson and Ikin 1946). (Note that in calculating the frequency of the O allele one must count not only people of group O with a double dose of the allele but also those people whose genotype is AO or BO.) From the above gene frequencies, the frequency of group O is 0.6827 × 0.6827, or 0.47 (47%); that of group AB is (0.2573 × 0.0600) × 2, or 0.03 (3%). The ‘×2’ is accounted for by the fact that A can combine with B, as well as B with A. In this book, unless otherwise stated, frequencies of alleles and phenotypes refer to a mainly white population in the UK. Frequencies for other populations are given by Mourant and co-workers (1976).
In different races the proportion of individuals belonging to a particular blood group varies widely; some examples of differences between Chinese, Europeans and West Africans are given in Table 3.3.
Table 3.3 Approximate frequencies (as percentages) of some blood group antigens in Chinese, Europeans and West Africans.
Several techniques are now available for determining the genotype of a polymorphic gene directly at the DNA level. These techniques are described in Chapter 8. The performance of these techniques is dependent on amplification of the relevant stretches of DNA, using the polymerase chain reaction (PCR) with appropriate primers. For a description of the PCR, see Chapter 16 and Plate 16.1.
Chromosomal Location of Different Blood Group Genes
The locations are: on chromosome 1, Rh, Fy, Sc, Cr and Kn; on 2, Ge; on 3 GLOB; on 4, MNSs; on 6, RHAG,I and HLA, genes for several complement components including C2 and the C4A and C4B markers Ch and Rg; on 7, Co, K, and Yt; on 9, ABO and GIL; on 11, In and Raph; on 12, Do; on 15, JMH; on 17, Di; on 18, Jk; on 19, H, Le, Lu, LW and Ok; on 22, P; and on the X chromosome, Xg, together with XK and the X-linked suppressor of Lu (mutated GATA 1, see Chapter 6).
Inherited Markers on Blood Cells and Predisposition to Disease
The relation between a genetic marker and disease may be expressed as linkage, i.e. the marker and disease susceptibility are inherited together, or as association, i.e. the particular marker is more frequent in subjects with the disease than in normal subjects.
The majority of HLA-associated diseases display a higher frequency of a particular HLA-DR antigen, for example in immunoglobulin A (IgA) deficiency and in several autoimmune diseases, the frequency of HLA-DR3 is increased (see Lechler 1994).
The first association between red cell groups and disease to be established was that between group A and carcinoma of the stomach (Aird and Bentall 1953), the risk of group A subjects being 1.2 times that of group O or B subjects (see review by Roberts 1957).
There is an association between group O and peptic ulcer (Aird et al. 1954), the risk for group O being 30–40% (1.3 times) higher than non-O (Mourant et al. 1978). There is also an association between non-secretors (of ABH) and peptic ulcer; non-secretors are almost 50% (1.5 times) more likely than secretors to have a duodenal ulcer (Clarke et al. 1956). The increased risk for group O and non-secretors is multiplicative, being about 2.5 times that of non-group O secretors (Doll et al. 1961). A partial explanation for these associations is that the Leb antigen (present only in secretors) is an epithelial receptor for Helicobacter pylori, a bacterium associated with gastritis, gastric ulcer and adenocarcinoma. Strains of the bacterium have a higher binding affinity (5-fold (median) higher) for OLeb molecules compared with ALeb (Borén et al. 1993, Aspholm-Hurtig et al. 2004). Individuals homozygous for non-secretor alleles are protected from infection by noroviruses, a common cause of acute gastroenteritis (Thorven et al. 2005). Individuals of group O once infected with cholera are more likely to have severe infections than individuals of non-O blood group (Harris et al. 2005) but have a selective advantage against severe malaria (Cserti and Dzik 2007; Anstee 2010; see also further discussion in Chapter 4).
An association of interest to haematologists is that between the levels of factor VIII, von Willebrand factor (vWF) and ABO group. Blood group O individuals have approximately 25% lower plasma levels of both factor VIII and vWF than individuals of group A,B or AB (Gill et al. 1987; O’Donnell and Laffan 2001). This variance may have an influence on the diagnosis of von Willebrand disease (vWd). Gill et al. found that 88 of 114 patients with type I vWd were group O and suggested that some individuals of group AB with a genetic defect of vWF may have their diagnosis overlooked because of the elevated levels of vWF in their blood group. Conversely, high levels of FVIII-vWF found in A,B and AB individuals may confer an increased risk of thrombosis and myocardial infarction (von Beckerath et al. 2004; Schleef et al. 2005, Tregouet et al. 2009).The higher levels of vWF in non-O individuals is likely a result of the increased half-life of vWF in non-O (25 h) versus O (10 h, Gallinaro et al. 2008).
Certain blood group phenotypes which are rare in most populations of the world are common in regions where malaria is endemic because they confer a survival advantage. In West Africa the Fy(a−b−) phenotype confers protection against Plasmodium vivax. In Central Africa the S−s− phenotype and in Papua New Guinea the Ge negative phenotype, protect against different strains of P. falciparum (discussed further in Chapter 6; reviewed in Anstee 2010).
Red Cell Markers on Functionally Important Membrane Structures
Some red cell antigens are situated on important structures on the cell membrane so that absence or presence of the antigen is associated with some structural abnormality of the cell, associated with functional change. Examples of such associations are as follows: (1) between Rhnull or Rhmod and haemolytic anaemia (see Chapter 5); (2) between the rare McLeod phenotype (see Kell blood group system, Chapter 6) and acanthocytosis; (3) between absence or depression of certain red cell antigens and elliptocytosis (see Chapter 6); (4) between Fy-related antigens and susceptibility to malaria (see Chapter 6); (5) between Cr-null (Inab phenotype) red cells and absence of decay-accelerating factor (see Chapter 6); and (6) between expression of the normally hidden antigen Tn and deficiency of sialic acid (see Chapter 7).
Effect of Neoplastic Change and Dyserythropoiesis on Blood Group Antigens
Cell Surface Antigens and Neoplastic Change
Neoplastic change in cells may be accompanied by changes in cell surface antigens. Some of these changes are due to incomplete synthesis of antigens normally present; others are due to abnormal synthesis, giving rise to neoantigens. As an example of the first kind of change, in some subjects with acute leukaemia, A, B and I antigens may be depressed (see Chapter 4); similarly, in some subjects with carcinoma, ABH determinants are lost due to incomplete synthesis, and precursor substances accumulate (Hakomori 1984). Loss of A,B and H antigens is frequently found in myeloid malignancies (Bianco et al. 2001). T and Tn antigens, which are normally covered by attached sialic acid, are commonly exposed on the surface of malignant cells (see Chapter 7).
The first recorded example of the production of a neoantigen by malignant tissue was that of Levine et al. (1951a). The patient was a woman with a gastric adenocarcinoma, whose serum contained the rare antibody anti-Tja (later renamed anti-PP1Pk). As expected, the patient’s red cells were Tj (a−) but a dried extract of the tumour specifically inhibited the antibody in her serum, suggesting that Tja antigen had been formed by the malignant tissue and might have evoked production of the anti-Tja. Thirty years later, biochemical analysis of lyophilized tumour tissue showed that glycolipids with P and P1 activity were present (Hakomori 1984). Similarly, some tumours derived from gastrointestinal tissue of group O or B individuals contain an A-like antigen, different from Forsmann antigen, and some tumours from Forsmann-negative tissue contain Forsmann antigen, which is similar to blood group A (Hakomori 1984). The overlapping functions of A and B transferases provide an explanation for the apparent expression of blood group antigens in certain tumours; changes in biochemical pathways in tumour tissue may provide appropriate substrates for the transfer of the ‘wrong’ sugars, giving rise, in subjects with exceptionally potent transferases, to A or B antigens not expected from the ABO genotype of the individual (Yates et al. 1983).
Various types of human adenocarcinoma accumulate a large quantity of fucolipids and their sialated derivatives as well as fucosylated glycoproteins with type II chains (see Chapter 4). Monoclonal antibodies directed against some of these structures have been produced and may prove to be useful diagnostic and therapeutic tools.
Dyserythropoiesis
In acquired dyserythropoiesis associated with a variety of haematological disorders (e.g. megaloblastic anaemia, sideroblastic anaemia) there is an increase in i and, in tests with some antisera, of I. In inherited (congenital) dyserythropoietic anaemia of type I and type II (HEMPAS), i is increased but I is probably not increased; see review by Worlledge (1977). Congenital dyserythropoietic anaemias (CDAs) are a rare group of inherited diseases in which erythropoeisis is altered quantitatively and qualitatively. Seven types of CDA are recognized by Wickramasinghe (1998). The clinical features of all types are reviewed by Delauney and Iolascon (1999) and Wickramasinghe and Wood (2005). CDA II (HEMPAS) is the most common and best characterized. Examination of bone marrow under the light microscope shows that about 10–40% of more mature erythroblasts are multinucleated. Under the electron microscope a double membrane is seen. This double membrane comprises the plasma membrane and elongated vesicles that run parallel and beneath the plasma membrane derived from endoplasmic reticulum (Alloisio et al. 1996). The causative mutations occur in the gene encoding secretory COP II protein SEC23B (Schwarz et al. 2009; Bianchi et al. 2009; see Chapter 6).
Hypoglycosylation of the major red cell anion transport protein is a characteristic but not exclusive feature of CDA II. Zdebska and colleagues (2001) report that glycosylation abnormalities of band 3, glycophorin A and glycolipids can be found in CDA I, II and III. These glycosylation abnormalities presumably account for the altered expression of I and i antigens reported in such cases. CDA I is characterized by the ‘Swiss-cheese’-like appearance of erythroblast heterochromatin on electron microscopy. Most patients with CDA I have mutations in the gene encoding codanin 1, but there is evidence that another gene may cause the disease in some cases (Ahmed et al. 2006).
CDA IV (formerly VII) is associated with severe transfusion-dependent anaemia and was first described in a Danish patient who had persistence of embryonic and fetal haemoglobins. The blood group phenotype of this patient [In(a−b−), AnWj–,Co(a−b−)] was remarkable and further investigation showed the red cells lacked CD44 and that aquaporin-1 was expressed at only 10% of normal levels (Agre et al. 1994; Parsons et al. 1994).This phenotype is most likely caused by a gain of function mutation (Glu325Lys) in the erythroid transcription factor EKLF (Singleton et al. 2009, reviewed in Singleton et al. 2012). Four patients with this phenotype have been described (Jaffray et al. 2013).
Myeloproliferative Disease
Myeloproliferative disease is thought to result from mutations in haemopoietic stem cells, giving rise to an abnormal clone of cells. Patients with polycythemia vera have a mutation in the cytosolic tyrosine kinase JAK 2 (V617>F) and their red cells demonstrate increased adhesion to laminin 10/11 resulting from increased expression and phosphorylation of the Lutheran blood group glycoprotein (Wautier et al. 2007). In several cases, Rh mosaicism has been observed; for example, in a patient whose probable genotype was originally DCe/dce, a proportion of the red cells typed as D negative (Mannoni et al. 1970). In another case, a man who had originally been D positive was found to have become D negative. An anomaly was found involving chromosome 1, on which the Rh genes are known to be located. The patient developed anti-D (and -C) following transfusion, demonstrating loss of immunological tolerance to self-antigens following the loss of ability to express these antigens (Cooper et al. 1979). In a further case, a woman with chronic myeloid leukaemia originally D positive became D negative and this was shown to result from a deleted RHD (Murdock et al. 2008).
Tn polyagglutination (Chapter 7), which is a form of mosaicism affecting red cells (and other blood cells), has also been observed in myelofibrosis (Bird et al. 1985).
Development of Red Cell Antigens
Red Cell Antigens in Embryos and Newborn Infants
During ontogeny, ABH and Lewis activity is at its highest from the fifth week after fertilization. ABH antigens are found in large amounts on endothelial cells, on most epithelial primordia and in practically all early organs, for example in the blood islands of the yolk sac, on digestive tube epithelia, etc. The central nervous system, liver, adrenal glands and secretory tubules show no activity at this stage. From the end of the 12th–14th weeks of gestation, there is regression of ABH expression from epithelial cell walls and from thyroid and other glands and organs (Szulman 1980). Similarly, although P1 is only weakly expressed on the red cells of newborn infants, on the red cells of embryos with a crown–rump length of less than 10 cm it is expressed almost as strongly as in the adult (Ikin et al. 1961). The reason for the regression of expression of these various antigens is unknown.
I, Lea, Leb and Sda are very weakly expressed on the red cells of newborn infants, and A, B, P1, Lua, Lub, Yta, Xg, Vel and Ch/Rg and antigens of the Cost collection are more weakly expressed on infants’ red cells than on adults’ red cells. The antigens of the Rh, K, Fy, Jk, MNSs, Di, Sc, Do, Co and Ge systems, as well as Aua of the Lu system, appear to be fully developed at birth.
Red Cell Antigens on Red Cell Precursors
Reticulocytes are as strongly agglutinated as mature red cells by anti-A and anti-B (Maizels and Paterson 1940; Winkelstein and Mollison 1965). Although A can be demonstrated even on early erythroblasts (Reyes et al. 1974; Gourdin et al. 1976), anti-A and anti-B do not inhibit the growth of colony-forming units (CFUs) or erythrocyte burst-forming units (BFU-Es) in vitro (Hershko et al. 1980) nor do they delay the engraftment of ABO-incompatible bone marrow in vivo (Dinsmore et al. 1983). The following red cell antigens have also been demonstrated on erythroblasts: A1, B and H (Yunis and Yunis 1963), I and those of the Rh, MNSs, P, Lu, K, Le, Fy and Jk systems (Yunis and Yunis 1964). The amount of D antigen on pronormoblasts is about one-quarter as great as that on mature red cells (Rearden and Masouredis 1977).
It seems that the onset of Hb synthesis and expression of glycophorins at the cell surface occur at the same stage of differentiation (Gahmberg et al. 1978). The temporal appearance of blood group antigens during erythropoiesis has been studied using in vitro culture of CD34+ cells derived from cord blood or adult peripheral blood in the presence of SCF, IL3 and EPO (Bony et al. 1999; Southcott et al. 1999). The earliest blood group markers expressed are ABO, Kell and the Rh-related glycoprotein RhAG followed by glycophorin A, band 3 and the Rh polypeptides. Lutheran appears late in the cultures.
Red Cell Antibodies
Antibodies are immunoglobulins (Igs), a family of proteins that are structurally related and all of which have two functions: to combine with antigen; and then to mediate various biological effects. Thus, all antibody molecules have antigen-combining sites and various effector function sites.
The common structural feature of all Ig molecules is an arrangement of a pair of identical, relatively large, ‘heavy’ (H) polypeptide chains joined, by covalent (disulphide) and non-covalent bonds, to each other and to a pair of identical ‘light’ (L) chains (Figure 3.2). The covalent bonds are mainly in the hinge region (see below). Five different classes of Ig are recognized, IgG, IgM, IgA, IgD and IgE, and these have different H chains, termed γ, μ, α, δ and ε. Igs of all five classes have the same L chains, although these may be either kappa (κ) or lambda (λ). In each IgG molecule, the two L chains are the same, e.g. a molecule may be γγκκ or γγλλ. IgG molecules occur as monomers, IgM molecules as pentamers, e.g. (μ2λ2)5 (see below), and IgA molecules as monomers or dimers. All Ig molecules have carbohydrate (CHO) attached to their H chains. For example, in IgG there is a short-branched CHO chain (approximately nine residues) attached to each H chain in the Cγ2 domain (see Figure 3.2). The CHO chains lie in the space between the two Cγ2 domains and may help to keep them apart. IgM has short CHO chains attached to each of the constant domains. The function of these CHO chains is not definitely known, although there is often loss of functional activity when the CHO is absent. In man there are four isotypes of IgG (IgG, IgG2, IgG3, IgG4) and two of IgA (IgA1, IgA2).
Figure 3.2 The IgG molecule. The constant (C) and variable (V) domains of the heavy (H) and light (L) chains are shown, together with the intra- and interchain disulphide bonds (i) that hold them together. The antigen-binding site is situated in the groove between the terminal parts of the VH and VL regions. The sites of attachment of carbohydrate chains indicated in the figure are, in fact, internal. After treatment with papain, the IgG molecule is cleaved into two Fab fragments and one Fc fragment; after treatment with pepsin, the two Fab fragments remain joined by disulphide bonds as an (Fab′)2 fragment and the Fc fragment disintegrates.
(Source: Adapted from Roitt 1989. Reproduced with permission of Elsevier).
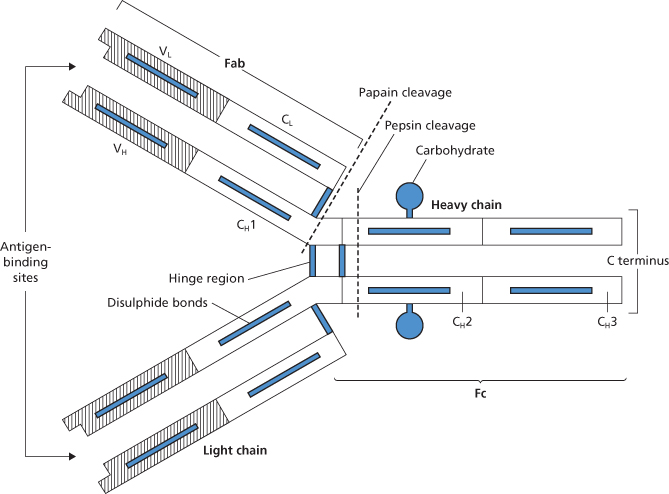
H and L chains are made up of regions (‘domains’), each of about 110 amino acids with the same basic structure. Both H and L chains take part in combining with antigen. The first 110–120 amino acids at the amino terminal end of each chain form the variable (V) domains, each of which contains hypervariable regions (four for VH and three for VL). The hypervariable regions on a pair of H and L chains, each consisting of fewer than 10 amino acids, come together in the folded molecule to form the antigen-binding site. The peptide sequences joining the hypervariable regions do not take part in binding to antigen but form an essential framework. The rest of the H and L chains are constant regions (CH and CL) with characteristic amino acid sequences. IgG and IgA have three constant regions per H chain but IgM and IgE have four. L chains each have only one C region. The CH1 domain of IgG antibodies (Cγ1 in Figure 3.3) plays a critical role in the assembly of the quaternary structure of IgG. CH1 will only fold correctly upon interaction with the light chain constant domain (CL) and is held in a reduced unfolded state by interaction with the chaperone protein BiP prior to interaction with the CL domain (Feige et al. 2009; Hebert and Gierasch 2009). There has long been a tendency to think of antibody molecules as having two distinct functional regions with antibody specificity conferred by the V domains and effector functions conferred by C domains. However, there is experimental evidence from studies of murine IgG indicating that amino acid sequence differences in the CH1 domain can influence the fine specificity and affinity of antibody molecules and suggesting that isotype switching is a mechanism for generating diversity in antigen binding (Torres et al. 2007). The different biological activities of each class of Ig molecule are affected by the amino acid sequences of the C regions. Flexibility is conferred on Ig molecules by a hinge region which, in IgG, is situated between the Cγ1 and Cγ2 domains (see Figure 3.2 and Figure 3.3) and in IgM in the CH2 domain (Figure 3.4). Flexibility is believed to be very important in enabling the molecule to fulfil its effector functions.
Figure 3.3 Structure of the four subclasses of IgG. The arrangement and number of the interheavy chain disulphide bonds are different in each of the subclasses. In IgG3 the hinge region is much longer than in the other subclasses.
(Source: Adapted from Roitt 1989. Reproduced with permission of Elsevier).
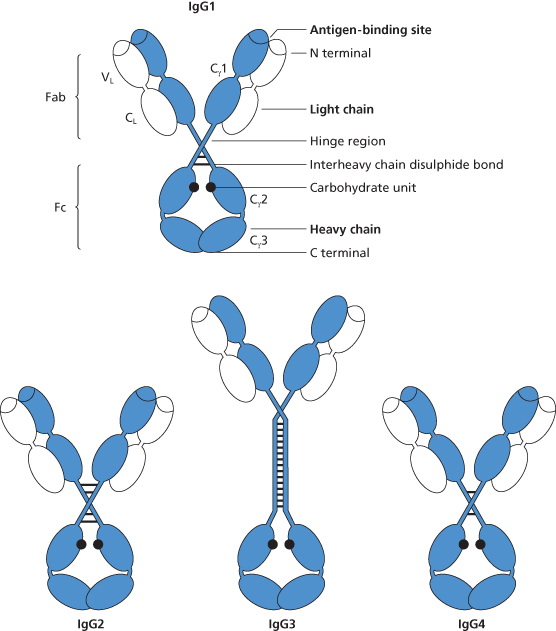
Figure 3.4 (a) The IgM molecule, showing the way in which the five subunits are held together by disulphide bonds and joined by joining, J, chain. As in Figure 3.1, the position of attachment of carbohydrate chains is shown, but their size and structure are not indicated. (b) Table form (as opposed to star form shown in (a) of IgM molecule). This configuration is observed when the molecule binds to a surface on which antigen sites are sufficiently close together to permit two or more antigen-combining sites of the same IgM molecule to bind (Czajkowsky and Shao 2009).
(Source: Adapted from Feinstein 1971. Reproduced with permission of John Wiley & Sons Ltd.)
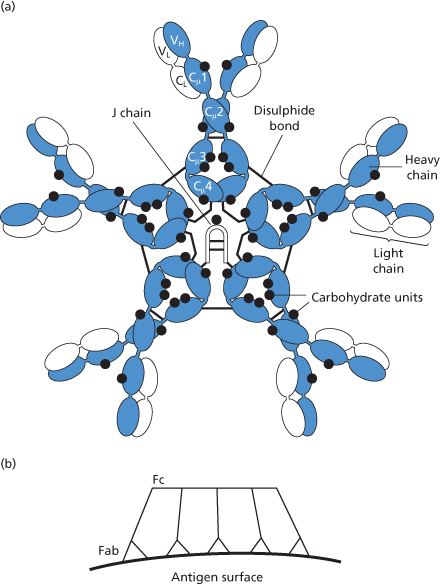
In IgG1 and IgG3, the site for binding C1q, and thus for activating the complement pathway (see later), is found on Cγ2, as is the site for binding to Fc receptors on mononuclear phagocytic cells. Sites on both the Cγ2 and Cγ3 domains are involved in binding IgG to Fc receptors on placental tissue. In IgM, the site for binding C1q is on the CH3 domains (see Figure 3.4).
By treatment with papain, the IgG molecule can be split, between the Cγ1 and Cγ2 domains (see Figure 3.2), into two Fab (fragment antigen-binding) fragments, each of which carries a single antigen-combining site, and one Fc (fragment crystallizable) fragment that carries sites for various effector functions (see above). As Figure 3.2 implies, the Fab fragment consists of the whole of the L chain and part of the H chain; in both chains, regions (VL and VH) at the amino terminal end of the fragment are joined to the single C region of the L chain and the Cγ1 region of the H chain. IgG can also be split into fragments by pepsin but in this case the molecule is cleaved nearer the carboxy terminal end (Figure 3.2) and the two Fab fragments are left joined together to form an F(ab′)2 fragment, the rest of the molecule being broken up (Porter 1967).
Characteristics of Different Immunoglobulins
The main immunoglobulins in serum are shown in Table 3.4.
Table 3.4 The main immunoglobulins in serum.
IgG
IgG is the most abundant Ig in plasma, having a mean concentration of about 12 g/l (range 8–16 g/l), so that the total plasma content, taking the plasma volume to be 40 ml/kg, is about 0.5 g/kg or, in a subject weighing 70 kg, 35 g; there is an approximately equal amount of IgG in the extravascular space, so that, in the example taken, total body IgG would be about 70 g, or 1 g/kg. The rate of transfer of IgG between the extra- and intravascular compartments is described briefly in Chapter 14.
Four subclasses of IgG are recognized and their mean concentrations in normal adult serum (in g/l) are as follows: IgG1, 6.63; IgG2, 3.22; IgG3, 0.58; IgG4, 0.46 (Morell et al. 1971). The subclasses differ in structure and function; thus, the number of inter-H-chain disulphide bonds varies in the different subclasses and, for example, IgG3 differs from the other subclasses in having a much longer hinge region (see Figure 3.3).
Several membrane-bound receptors recognize the Fc region of antibodies. FcRI, FcRII and FcRIII recognize sites on the lower hinge region (CH2) of IgG (Sondermann and Oosthuizen 2001) (Colour Plate 3.3). Monomeric IgG1 and IgG3 bind to FcRI; polymeric IgG1 and IgG3 bind, in addition, to FcRII and FcRIII. Three closely linked genes encode three different types of FcRII: FcRIIa, FcRIIb and FcRIIc. FcRIIa has two variants, one binds only weakly to IgG2 but the other, present in 30% of white people, binds strongly (Warmerdam et al. 1991). IgG4 binds weakly to both forms of FcRIIa. Glycosylation is essential for some functions of IgG, including adherence to Fc receptors.
IgG is the only Ig to be transferred across the placenta from mother to fetus, a fact that explains the role of IgG antibodies in the aetiology of alloimmune cytopenias of the fetus and newborn. The transfer of IgG across the placenta from mother to fetus is mediated by the neonatal Fc receptor (FcRn) (see Chapter 12 and Plate 12.1). FcRn has a different structure from the other Fc receptors and consists of a heavy chain complexed with beta2 microglobulin (Burmeister et al. 1994).
IgG1 and IgG3 activate complement strongly but IgG2 activates only weakly and IgG4 not at all. IgG1, IgG2 and IgG4 myeloma proteins have catabolic rates that are similar to those of total IgG, i.e. they have a t1/2 of about 21 days but the t1/2 of IgG3 is about 7 days (Morell et al. 1970). The foregoing estimates were derived from measurements on myeloma proteins. Similar half-lives have been found for monoclonal Rh D antibodies, namely for IgG1, 21–22 days (Callaghan et al. 1993; Goodrick et al. 1994) and for IgG3, 10 days (Goodrick et al. 1994).
IgM
The plasma concentration of IgM is 0.5–2.0 g/l; about 74% of total body IgM is intravascular; the plasma t1/2 is 5 days. IgM molecules are held together by a J (joining) chain (see Figure 3.3).
IgM is more effective than IgG in activating complement. The difference is due to the fact that C1 is activated only when it is attached by two of its C1q heads to the activating molecule. Thus, whereas a single bound IgM molecule can activate C1, at least two IgG molecules bound at closely adjacent sites are needed. The structure of IgM is depicted in Figure 3.4a and a model of the 3-dimensional structure showing the position of amino acid residues believed to directly interact with C1q is shown in Colour Plate 3.4. When IgM molecules bind to antigen a table-like structure is formed with all Fab domains bent toward the protruding central portion formed by the C-terminal domains (Czajkowsky and Shao 2009; Figure 3.4b).
IgA
The plasma concentration of IgA is 1.4–4.0 g/l; about 40% of total body IgA is intravascular; the plasma t1/2 is 6 days.
IgA is the only Ig present in epithelial secretions. Whereas, in plasma, IgA is predominantly monomeric, in secretions it occurs mainly as dimers, which are held together by a J chain. In secretions, IgA has a ‘secretory piece’ that prevents the molecule from being digested. The presence of IgA in secretions is believed to be due almost entirely to local production rather than to transport from plasma. The function of IgA is evidently to neutralize antigens that might otherwise enter the body via mucosal routes.
There are two subclasses of IgA, a major component, IgA1, and a minor component, IgA2, which has two serologically distinguishable variants, A2m(1) and A2m(2). Both IgAl and IgA2 bind to an Fc receptor for IgA (CD89) on monocytes and macrophages. Inhibition of IgA binding to CD89 by staphylococcal superantigen-like proteins leads to increased survival of staphylococcal bacteria in blood (Langley et al. 2005).
IgD and IgE
Both of these Igs act mainly as cell receptors. No free antibodies made of IgD have been described, although IgD is synthesized by, and demonstrable on, the surface of B lymphocytes and is probably involved in the activation of these cells by antigen.
IgE is synthesized by plasma cells and is present in the plasma in very low concentrations. It has a very high affinity for Fc receptors (FcεRI) on basophils and mast cells. The binding of antigen to IgE antibody on the surface of these cells leads to crosslinking of receptors and produces degranulation with release of vasocative amines, such as histamine, and of cytokines as well as synthesis of inflammatory mediators of arachidonic acid (e.g. leukotrienes). These mediators are responsible for producing such atopic phenomena as asthma and hay fever. The role of IgE antibodies in reactions to atopens is discussed in Chapter 15.
Markers on Immunoglobulin Molecules
Idiotypic Markers
Idiotypic determinants arise from the unique configuration of the antigen-combining site of an antibody molecule, which gives the molecule its specificity.
The idiotype of a particular antibody specificity is composed of several individual determinants called idiotopes, in much the same way that the Rh polypeptides expressed by the R1 haplotype encompass several Rh determinants (i.e. D, C, G, e, Rh17, Rh18, Rh29, Rh34, Rh44, Rh46, Rh47, etc.). Some of the individual idiotopes arise from the unique amino acid sequences in the hypervariable region that come into contact with the antigen in the antibody-binding site; others arise from framework amino acid sequences. Antisera can be raised to both sets of idiotopes.
Some idiotypes (public idiotypes) are common to all antibodies of a single specificity; each individual can make 106–107 different antibody specificities. Other idiotypes (private idiotypes) are restricted to only a few clones producing antibodies of similar specificity but each differing slightly (by one or two amino acids) in their hypervariable region; a typical polyclonal immune response can be composed of 104 different species of antibody molecules of similar specificity, each species being the product of a separate B cell clone. Sometimes, antibodies of quite distinct specificities, for example anti-albumin and anti-influenza virus can exhibit common idiotypes; these are called the major crossreactive idiotypes and represent common amino acid sequences in the framework regions of their binding sites.
Every antibody is capable of inducing the formation of auto- or allo-anti-idiotypes. The formation of auto-anti-idiotypes is believed to be important in regulating the immune response.
Allotypic Markers
These are antigens, found on γ, α and κ chains, the inheritance of which is controlled by alleles of the gene coding for a particular Ig chain. For example, H chains of IgG subclass 1 can carry either G1m(z) or G1m(f), the difference being determined by a single amino acid substitution. Further details are given in Chapter 13.
Isotypic Markers
These are antigenic determinants on H chains and on certain L chains, which define and characterize the class or subclass of Ig molecules and which are sequences on the C region of γ and μ chains or on the C region of κ and λ chains. Isoallotypic markers (formerly called non-markers) are those which are isotypic markers for one subclass but are allotypic markers in another subclass. For example, G4m(a) is an allotype in subclass IgG4 but is an isotype in IgG2.
Immunoglobulins in Fluids Other Than Plasma
Immunoglobulins are found in the following fluids.
Colostrum and Saliva
In colostrum, the IgA concentration is about 90% and in saliva is about 20% of that in normal serum (Chodirker and Tomasi 1963). By contrast, concentrations of IgG and IgM in colostrum are respectively 1% and 5–10% of the amounts in normal serum, and in saliva only faint traces of IgG and IgM are present (Adinolfi et al. 1966). The titre of IgA antibody tends to be higher in colostrum than in serum (Tomasi et al. 1965; Adinolfi et al. 1966). Colostrum frequently contains potent IgA anti-A and anti-B, but in saliva these antibodies are usually present only in low concentration (see Chapter 4).
Ascitic Fluid
Several alloantibodies have been harvested from ascitic fluid; anti-Yta (Eaton et al. 1956); anti-c and -Lea (MM Pickles, quoted by Race and Sanger 1968, p. 255); and anti-E (Zeitlin et al. 1958) and anti-K (Longster and Major 1975). In the last mentioned case the titre of the antibody was about the same in serum as in ascitic fluid and the antibody was probably IgG; in contrast to that of anti-K, the titres of anti-A and anti-B were slightly lower in ascitic fluid than in serum.
Amniotic Fluid
The IgG concentration at or near term is about 0.1–0.2 g/l, i.e. 1/50–1/100 of that in normal adult serum.
Production of Immunoglobulin in the Fetus and Infant
Changes in Ig levels in the first year of life are summarized in Figure 3.5.
Figure 3.5 Concentration of IgG, IgM and IgA in the first year of life, as percentages of the normal adult levels. The dotted line indicates IgG derived from the mother by placental transfer.
(Source: Based on the data of West et al. 1962.)
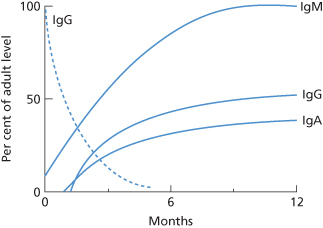
IgG
Although most of the IgG in the serum of newborn infants is derived from the mother by placental transfer, a small amount is of fetal origin as shown by the fact that it has the father’s Gm allotype (Mårtensson and Fudenberg 1965).
The time at which the synthesis of substantial amounts of IgG begins after birth was studied by Zak and Good (1959) in two normal infants born to mothers with severe hypogammaglobulinaemia. The IgG level, which was negligible at birth, started to increase between 3 and 6 weeks; antibodies were first demonstrable at about 2 months, by which time the IgG level had reached 2 g/l. At 10–18 months, IgG levels are about 60% of adult values (Buckley et al. 1968).
IgM
IgM is not transferred across the placenta. The concentration in cord serum is between 5% and 10% of that found in adult serum. IgM antibodies of various specificities can be found in cord serum: anti-I in most infants (see Chapter 7); anti-A and anti-B in occasional infants (see Chapter 4); anti-Gm in most infants (see Chapter 13) and anti-A chain in about 10% of infants (Epstein 1965). Within 2 or 3 days of birth the concentration of IgM starts to rise and reaches 50% of the adult level at 2–3 months and 100% at about 9 months; between the ages of 9 months and 3 years values remain at the adult level, although between 5 and 9 years they are at the lower end of the adult range (West et al. 1962).
IgA
This protein cannot be detected in cord serum. By the age of 2 months, the amount in the serum has reached about 20% of the adult level (West et al. 1962) and by the age of 5 years about 50% (Buckley et al. 1968).
Immunoglobulin Class and Subclass of Red Cell Alloantibodies
Red cell alloantibodies may be naturally occurring or immune. When naturally occurring they are most often IgM but may be partly IgG or, occasionally, predominantly IgG (see also p. 80); when immune they are most often IgG, but may be IgM or a mixture of IgM and IgG; they are sometimes partly IgA. Cells derived from a single clone make antibodies of the same specificity but any given cell can make only one class of antibody.
Determination of the subclass of antibodies is difficult. There is more than 95% sequence homology between most of the C regions of human IgG subclasses. There are therefore few subclass-specific epitopes and it is difficult to raise antibodies against them (Michaelsen and Kornstad 1987). If insufficiently absorbed sera are used, false-positive results are obtained. If well-absorbed sera are used, some antibodies fail to react with all subclass sera.
Investigations of the subclass composition of different blood group antibodies are referred to in the three following chapters.
Immunoglobulins on B Lymphocytes
The Ig on the surface of lymphocytes gives them the capacity to react with an antigen of corresponding specificity and to develop into active antibody-secreting cells. The two major classes of Ig demonstrable on the surface of immature B lymphocytes are IgM and IgD; the latter appears to have a role in triggering the cell’s response to antigen.
In subjects immunized to Rh D, anti-D-bearing lymphocytes can be demonstrated by incubating D-positive red cells with the blood of the immunized subjects and observing ‘rosettes’, i.e. single lymphocytes surrounded by many adherent erythrocytes. Rosetting could be demonstrated in two out of eight women immunized by pregnancy and in five out of five hyperimmunized volunteers following a booster injection of red cells; in these latter subjects the number of rosettes increased to a maximum on about the 10th day after re-stimulation (Elson and Bradley 1970).
The Ig Superfamily
The name Ig superfamily is given to all those molecules that contain domains within their structures that are related to either the variable or constant domains of immunoglobulins. Most of the molecules concerned play an important role at the cell surface in cell–cell adhesive interactions or in cell–extracellular matrix interactions. The Ig superfamily includes the antigen receptors, i.e. immunoglobulins and T-cell receptors, Fc receptors, as well as several other lymphocyte cell surface molecules, such as CD4 and CD8 and MHC class I and II molecules. It also includes several blood group active molecules on red cells (Lutheran, LW, Oka; see Chapter 6).
Methods of Separating and Identifying Immunoglobulins
IgG can be affinity purified with particle-bound bacterial proteins A and G. Protein A and Protein G although structurally distinct both bind to the Fc portion of antibody molecules at the interface of the CH2 and CH3 domains (Sauer-Eriksson et al. 1995). Protein A binds human IgG1, IgG2 and IgG4 but not IgG3.
Immunoglobulins may be separated according to their different charges, for example by ion-exchange chromatography or by their different sizes, i.e. by passage through a ‘molecular sieve’ as in gel filtration.
The greater positive charge on IgG compared with other plasma proteins is exploited in one method of preparing anti-D IgG for immunoprophylaxis, IgG being separated from plasma on a diethylaminoethyl (DEAE)-cellulose column (Hoppe et al. 1973). This method of separating IgG from plasma has a much higher yield than that of cold-ethanol precipitation.
Some IgG antibodies can be separated from one another by fractionation on carboxymethyl (CM) cellulose or hydroxyapatite columns.
Anti-D, and some other red cell alloantibodies, are more positively charged than the bulk of IgG in the serum and can thus be partially separated from it. Similarly, the charge on different alloantibodies varies. Examples of separation of anti-D from the bulk of IgG and of other alloantibodies from one another, on carboxymethyl-cellulose columns, are shown in Figure 3.6.
Figure 3.6 Distribution of the total IgG (– – – –) and of particular antibodies (––––) in fractions eluted from carboxymethyl-cellulose (using buffers or increasing molarity), expressed as percentages of the total amount recovered from the columns. The four small figures show the distribution of single antibodies in four sera; the larger right-hand figure shows the elution of two antibodies from one serum.
(Source: Frame and Mollison 1969. Reproduced with permission of John Wiley & Sons Ltd.)
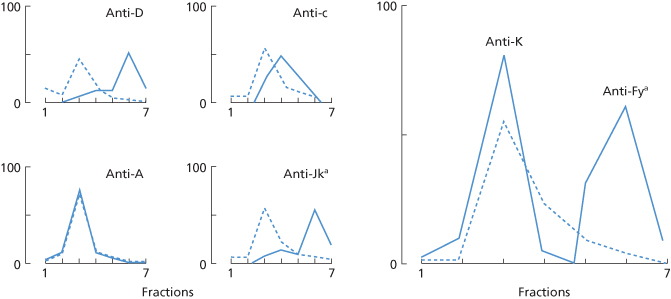
Identification of Immunoglobulins Using the Antiglobulin Test
Determination of the Ig class of red cell antibodies is most conveniently performed using Ig class-specific antiglobulin reagents (Polley et al. 1962; Adinolfi et al. 1966).
Effect of Reduction on Different Immunoglobulins
Effect on IgM
Mild reduction of human IgM with sulphydryl compounds cleaves intersubunit (IgMs–IgMs), intersubunit-J chain and intrasubunit (H–H, H–L) disulphide bonds. Intersubunit bonds are more sensitive than intrasubunit bonds to reduction. Mild reduction releases J chain from the IgM molecule.
Treatment of serum with a reducing agent abolishes the agglutinating activity of most IgM antibodies. IgM subunits of anti-D, anti-A and anti-B retain their ability to combine with red cell antigens, but subunits of IgM anti-Lea have no serological activity, indicating that their binding affinity is very low (Holburn 1973).
If a monoclonal IgM is mildly reduced and then dialysed against saline to remove the reducing agent, the subunits may slowly reassemble, if the suspending medium does not contain non-specific IgM. It would therefore be expected that if a purified IgM antibody, or for example an eluate, were treated in the same way, the serological activity of the antibody would be partially restored. On the other hand, when an IgM antibody in whole serum is mildly reduced and the serum is then dialysed, restoration of serological activity is not expected, because the subunits of IgM reassemble at random and most of the IgM molecules in the serum are of different antibody specificities.
The reassembly of IgM subunits to form 19S IgM can be prevented by treatment with alkylating agents, such as iodoacetamide (IAA). Such agents irreversibly block the SH groups which have been liberated by reduction of the protein, as well as any free SH groups originally present.
Effect on IgG
After mild reduction, some IgG incomplete antibodies such as anti-D become weakly agglutinating (Chan and Deutsch 1960; Romans et al. 1977). The changes in the properties of the IgG molecule are brought about by the breaking of the disulphide bonds in the hinge region of the molecule, permitting the two antigen-binding sites to move further apart and thus bridge the distance between red cells. The two halves of the molecule remain held together by strong non-covalent bonds between the CH3 domains (see Figure 3.2). Reduced IgG3 anti-D monoclonals are more potent direct agglutinators than reduced IgG1 anti-D monoclonals (Scott et al. 1989).
The modification of the properties of the IgG molecule produced by mild reduction are stabilized by treatment with alkylating reagents (e.g. IAA).
Practical Applications
Treatment of serum with the reducing agents 2-mercaptoethanol (2-ME), dithiothreitol (DTT) or its isomer dithioerythritol (DTE), is commonly used in blood group serology to distinguish between IgG and IgM antibodies as, if the agglutinating activity is abolished, it is virtually certain that the antibody in question is IgM.
DTT is preferred to 2-ME for the following reasons: first, it is more efficient in maintaining reduction; second, it is more resistant to oxidation in air; and third, it lacks an offensive odour.
Serum that has been treated with 2-ME may be tested for agglutination without preliminary dialysis (Reesink et al. 1972), although if the mixture of undiluted serum and 2-ME is tested by the indirect antiglobulin test, false-positive reactions occur (Freedman et al. 1976). False-positive reactions also occur occasionally if DTT-treated undiluted serum is left with red cells for more than 2 h. False-positive reactions are not observed when dilutions of 2-ME-treated or DTT-treated serum are tested.
Heat Lability of Different Immunoglobulins
Heating at 56°C for 3 h has little effect on anti-D (IgG) but produces a just detectable fall in anti-P titre (IgM). Heating at 63°C for 3 h decreases the IgG anti-D titre by about one-third, whereas heating at 63°C for only 1 h almost completely inactivates anti-P1 (Adinolfi 1965). IgA antibodies, like IgG antibodies, are unaffected by heating at 56°C for 3 h (Adinolfi et al. 1966).
Naturally Occurring Antibodies
In blood group serology, the term naturally occurring is used for antibodies (Table 3.5) found in the serum of a subject who has never been transfused or injected with red cells containing the relevant antigen or been pregnant with a fetus carrying the relevant antigen.
Table 3.5 Naturally occurring alloantibodies to red cell antigens.
System (or antigen) | Specificity of antibodies | Subjects in whom antibodies occur |
ABO/Hh | A, B, H | All without corresponding antigen |
A1 | 1–8% of A2 | |
22–35% of A2B | ||
HI | Occasional A1 and A1B | |
Lewis | Lea | 20% of Le (a–b–), ABH secretors |
Leb | Often accompanies anti-Lea; rare on its own | |
P/P1 | Pk, PP1Pk | All without corresponding antigen |
P1 | Common in P2 | |
I | I | Most i |
MNSs | M | 0.02% of M–N+ |
N | 0.002% of M+N– | |
S | Very rare in S– | |
VW, Mg | 1–2% of population | |
Rh | E | 0.1% of D positives |
C, Cw, Cx | Very rare | |
D (cold) | 0.15–0.3% of D negatives | |
Sd | Sda | 1–2% of population |
Di | Wra | 1% of population |
Ge | Rare | |
Kk | K | Rare |
Lu | Lua | 0.02% of population |
The list is incomplete: specificities that have been found occasionally include anti-Ina, -Lub, -LW and -Yta amongst others; naturally occurring antibodies to several low-frequency antigens other than Vw, Mg and Wra are common; the figures given for frequencies are only approximate; further details are given in the relevant chapters on blood groups.
Landsteiner (1945) concluded that natural antibodies had a dual origin, antigen induced and spontaneous. As examples of the first kind, anti-A and -B may be cited. These antibodies are believed to be heteroagglutinins produced as a response to substances in the environment, which are antigenically similar to red cell alloantigens (Wiener 1951).
A direct demonstration that anti-B titres can be increased in humans by the ingestion or inhalation of suitable bacteria was provided by Springer and Horton (1969). In both infants and adults, the oral administration of killed E. coli O86, with blood group B specificity, produced rises in anti-B titre in a proportion of subjects; responses were commoner in subjects with an intestinal disorder, suggesting that antigen was absorbed more readily when the mucosal surface was damaged. Four out of 12 adults showed an increase in anti-B titre after receiving a nasal spray of E. coli O86. Similar observations have been reported for anti-T and anti-Tn (Springer and Tegtmeyer 1981). These observations raise the question of how the proliferation of E. coli O86 is controlled in the gut of Group B individuals. An explanation is provided by Stowell et al. (2010) who show that the carbohydrate binding proteins galectins 4 and galectin 8 which are highly expressed in gastrointestinal mucosa are able to effect killing of E. coli O86. These authors postulate that galectins provide protection from blood group-active pathogens irrespective of the ABO group of the host and speculate that the existence of galectins may have allowed the evolution of the ABO blood groups.
Other antibodies which may be heteroagglutinins include anti-H, -PP1pk and -Pk, found in virtually all subjects who lack the corresponding antigen, and anti-A1, -HI, -Lea and -P1, found in only some subjects who lack the corresponding antigen. In some cases, the formation of anti-K has evidently been due to infection with a microorganism producing a K-like substance.
There is abundant evidence that some natural antibodies are spontaneous, i.e. generated without the intervention of antigens: natural antibodies are present in newborn nude, germ- and antigen-free mice, sometimes in higher proportions than in normal mice (Avreamas 1992).
Examples of red cell antibodies that are probably spontaneous in origin are anti-E and various others in the Rh system (see Chapter 5), antibodies to low-incidence antigens, and anti-Lua, -Dia and -Xga (see Chapter 6) and anti-HLA, found in about 1% of normal donors (see Chapter 13). In autoimmune haemolytic anaemia, it is common to find several antibodies to low-incidence antigens, for example to Wra, Swa, Cx, Mia and Vw, to which the patient has clearly not been exposed (Cleghorn 1960).
Naturally Occurring Antibodies and Class of Immunoglobulin
Most, but by no means all, naturally occurring antibodies are cold agglutinins (IgM) and immune antibodies of the same specificity are either warm IgM or IgG antibodies.
Anti-A and anti-B are always partly IgM and may be wholly IgM; in group O subjects anti-A and -B are always partly IgG and may be partly IgA. Anti-A1 in A2 and A2B subjects seems always to be wholly IgM, but anti-A1 separated from group O serum may be only IgG (see Chapter 4). Examples of anti-HI and -H have been solely IgM (Adinolfi et al. 1962; Chattoraj et al. 1968).
Although most examples of anti-Lea behave on ordinary serological testing as if they were solely IgM, an IgG component can often be demonstrated by appropriate methods. Rare examples of anti-Lea and -Leb are solely IgG. Naturally occurring antibodies of the MNSs system may be IgM or IgG.
In the past, many examples of naturally occurring anti-E were considered to be solely IgM because they gave a negative indirect antiglobulin test but, with the introduction of more sensitive methods, an IgG component is usually detectable and some examples may be solely IgG. Rather surprisingly, cold-reacting anti-D, demonstrable in the AutoAnalyzer, has been found to be IgG. References supporting the foregoing statements and further details will be found in Chapters 4–6.
Naturally occurring antibodies to low-frequency antigens (e.g. anti-Wra) are mainly IgM but a large proportion of them have an IgG component and some are solely IgG (Lubenko and Contreras 1989).
Immune Responses to Red Cell Antigens
Immune responses to foreign antigens are mediated by lymphocytes. There are two types of immune response, humoral and cellular. In the humoral response, antigen is recognized by receptors on T cells, which subsequently stimulate B lymphocytes. The T-cell receptors are extremely diverse and are capable of recognizing all of the foreign molecules that may be encountered. A few antigens can stimulate B cells directly. Stimulation of B lymphocytes results in their proliferation and, eventually, in the production of plasma cells. Some of the primed B cells do not differentiate and remain, in the absence of further antigenic stimulation, as memory cells. On re-stimulation, memory cells mount a secondary (anamnestic) response; this response is characterized by the rapid production of antibody that is more potent and specific than that produced in the primary response.
Antigen Recognition
Before a humoral response can be mounted, antigen must be brought into contact with antibody-forming cells, i.e. B lymphocytes. The way in which this is done and the role of HLA antigens in the process are described in Chapter 13.
Primary and Secondary Responses to Red Cell Antigens
Primary responses to any particular antigen can, by definition, be studied only in subjects who have not previously encountered the antigen. In the case of some antigens, for example A and B, it can be presumed that there has been previous exposure, as antigens identical with or similar to the human ones are found in a wide variety of organisms, and in the case of others, for example Lea, P1, previous exposure to the antigen is also possible (see Chapter 4). Although it is difficult or impossible to study primary responses to these antigens, such responses can be studied in many other systems, for example Rh, K, Fy and Jk, in which naturally occurring antibodies are rare. As Rh D is the most immunogenic of the latter antigens, it has been used in most of the systematic work that has been done. As described in Chapter 5, after a first injection of 1 ml of D-positive red cells to D-negative subjects who have not previously been exposed to the antigen and who have no trace of anti-D in their serum, antibody can first be detected in some subjects after about 4 weeks; in these subjects, the concentration of antibody increases slowly and after 6–10 weeks reaches peak values not exceeding about 4 µg/ml (Samson and Mollison 1975; Contreras and Mollison 1981). In other subjects, antibody can first be detected only after two injections, given at an interval of 3 months or more; less commonly, antibody is first detected only after three or more injections. There is evidence that in all subjects in whom antibody is ultimately detected, primary immunization is induced by the first injection of red cells because in such subjects red cells injected on the second occasion invariably have a shortened survival (see Chapter 10). There are some subjects in whom no antibody is formed even after many injections given over a period of 2–3 years, and these subjects are classified as non-responders. In these subjects, D-positive red cells have a strictly normal survival even after many injections of D-positive red cells have been given.
The production of anti-D after a first injection of D-positive red cells cannot be hastened by injecting a much larger amount of red cells, although the number of responders increases, as does the amount of antibody produced in the primary response (see Chapter 5).
It is known that in animals, after a first injection of antigen has been given, there is a certain period during which the animal will not respond well to a second injection. For example, in horses injected with tetanus toxoid, the period was at least 3 months, although less than a year (Barr and Glenny 1945). In humans, the number of D-negative subjects forming anti-D within 6 months did not appear to be greater when injections were given every 6 weeks than when only a single injection was given (Archer et al. 1969).
In subjects already immunized to Rh D, following a (further) transfusion of D-positive red cells, the concentration of anti-D in the serum starts to increase about 3 days after transfusion and then rises logarithmically to reach a peak value, which may be as high as about 1000 µg/ml (i.e. 10% of the total IgG), some 10–20 days later (see Chapters 5 and 11). The response to a first injection of group A or B red cells in a group O subject is similar in that antibody concentration rises rapidly and reaches a peak within about 12 days (see Figure 4.1 and Figure 4.2), indicating that these responses should be regarded as secondary.
The differences between primary and secondary responses to the D red cell antigen in humans are very similar to those between primary and secondary responses to bacterial toxins in animals (Burnet and Fenner 1949).
There have been few systematic studies of immune responses to alloantigens other than D; the production of serologically detectable anti-K, -E and -C within 4 weeks of a first transfusion has been reported in children with burns (Bacon et al. 1991). The production of anti-Jka and anti-P1 within 2 weeks of a first transfusion has been reported in a 7-year-old child (Cox et al. 1992).
Class of Immunoglobulin Produced in Red Cell Alloimmunization
In the primary response it is usual for IgM antibody to be formed initially and for production then to be switched to IgG antibody, but it has proved difficult to confirm that this generalization applies to the production of red cell alloantibodies in humans. For example, in investigating responses to the Rh D antigen, using manual tests, anti-D can often first be detected by the agglutination of enzyme-treated red cells at a time when the indirect antiglobulin test is negative. As it is known that both IgM and IgG anti-D in low concentrations will agglutinate enzyme-treated cells, and as all serological reactivity is usually lost if attempts are made to fractionate serum containing such antibodies, it is usually impossible to decide the Ig class of the antibody present.
Although it is not known with certainty whether IgM antibody is the first to be made in subjects responding to the Rh D antigen, it is quite clear that in the majority of subjects IgG antibody soon predominates and is often the only type that can be identified at any time. In a minority of subjects IgM antibody is also produced in substantial amounts. In hyperimmunized donors anti-D is also quite often partly IgA (see Chapter 5).
Responses to several other red cell antigens, for example K, Fya and Jka, appear to be similar to responses to D; that is to say, most antibodies are predominantly IgG, although in some subjects a mixture of IgM and IgG antibodies is found. Lutheran antibodies may sometimes be partly IgA (see Chapter 6).
Blood group systems in which naturally occurring antibodies are found demand separate consideration. In the ABO system perhaps all subjects should be regarded as immunized. Moreover, ABO-incompatible pregnancies and injections of various animal products cause both quantitative and qualitative changes in anti-A and anti-B (see Chapter 4). Perhaps the most interesting fact about the production of ABO antibodies is that immune anti-A and anti-B are predominantly IgM in A or B subjects but may be largely IgG (and partly IgA) in group O subjects.
Alterations in Binding Constant
IgG antibody formed late in the immune response tends to have a higher binding constant than antibody formed early in the response; for example, an increase in the binding constant of anti-D during the secondary Rh D immunization was demonstrated by Holburn et al. (1970).
Increased Heterogeneity of Antibody in Hyperimmunized Subjects
There is evidence that as immunization progresses the antibody tends to be more diverse with regard to Ig class and subclass. Data with regard to anti-D are given in Chapter 5.
Persistence of IgM and IgG Antibodies
IgM antibodies tend to decline rapidly in concentration after the last stimulus and usually become undetectable after 1–2 years. Some IgG antibodies (e.g. antiRh D) decline far more slowly and may be readily detectable 30 years after the last stimulus (see Chapter 5); others (e.g. anti-Jka) may become undetectable a few months after the last stimulus. In a follow-up with a median period of 10 months, of 160 patients in whom 209 antibodies had been detected, some of the findings were as follows: of 39 antibodies belonging to the Lewis, MN or P1 systems (presumably IgM), 28 became undetectable, whereas of 170 belonging mainly to the Rh, K, Fy and Jk systems, which were presumably IgG, only 49 became undetectable. Antibodies of the Kidd system became undetectable more frequently than those of the Rh system, in 11 out of 21 compared with 27 out of 98, although this difference may have been partly due to the relative potency of the antibodies concerned; of antibodies (of all specificities) with initially weak reactions, 41 out of 84 became undetectable whereas of those with initially very strong reactions, only 1 out of 18 became undetectable (Ramsey and Larson 1988). In a retrospective study, 5–10 years after first being identified, 14 out of 36 alloantibodies could no longer be detected on at least one occasion. Of a further 22 antibodies looked for after more than 10 years, only 10 could still be detected (Ramsey and Smietana 1994).
Relation between Immunoglobulin Class of Antibody and Serological Behaviour
IgM Antibodies
Most IgM antibodies will agglutinate red cells suspended in saline. Agglutination by some IgM antibodies, for example anti-A (Polley et al. 1963) is not enhanced in a medium of serum whereas that of some other antibodies is. Using purified IgM anti-D, it was found that the agglutinin titre was greater by four or five doubling dilutions when using serum rather than saline as a diluent; the effect was observed with serum diluted up to 1 in 32 (Holburn et al. 1971a). The enhancing effect of serum is, therefore, important only when comparing sera with titres of more than 32 or when determining the titre of purified antibodies.
The titre of IgM antibodies is about four times higher with enzyme-treated red cells than with untreated cells (Aho and Christian 1966; Holburn et al. 1971a). Evidently, very weak IgM antibodies may be detectable only with enzyme-treated red cells.
Naturally occurring IgM antibodies of the ABO, Lewis and P systems agglutinate red cells more strongly at 0°C than at higher temperatures. For example, the titre of anti-A and anti-B is about eight times higher at 0°C than at 37°C (Kettel, cited by Wiener 1943, p. 19). Although anti-A and -B almost invariably agglutinate appropriate red cells at 37°C, other antibodies of the aforenamed systems, for example anti-A1, -HI, -Lea -Leb and -P1, do not usually agglutinate red cells above 20–25°C; occasional examples of these antibodies will agglutinate up to about 30°C and even give trace reactions at 37°C; examples with such a wide thermal range as this will invariably bind complement. The behaviour of anti-Lea is slightly different in that although it will usually not agglutinate cells above a temperature of about 20–25°C, it will usually bind to red cells and fix complement at 37°C and thus give a strongly positive indirect antiglobulin test.
Anti-D agglutinins produced in an immune response are ‘warm’, i.e. they are as active at 37°C as at lower temperatures (Levine et al. 1941). A few IgM antibodies are ‘incomplete’, for example occasional examples of anti-Jka (Adinolfi et al. 1962; Polley 1964).
IgG Antibodies
In most blood group systems IgG antibodies will not agglutinate untreated red cells suspended in saline; such non-agglutinating antibodies used to be described as ‘incomplete’.
Potent examples of IgG anti-D either in undiluted serum, or in some cases in serum diluted 1:2 in saline, will agglutinate saline-suspended red cells (Hopkins 1969, 1970). In fact, it has subsequently become clear that really potent IgG anti-D, even at a dilution of 1 in 10 or more, will agglutinate saline-suspended cells (unpublished observations, M. Contreras). Potent IgG anti-K and anti-M may also agglutinate saline suspended red cells.
IgA Antibodies
IgA fractions containing anti-A and anti-B will agglutinate red cells suspended in saline; the titre is increased about four-fold by using the indirect antiglobulin test with an anti-IgA serum (Adinolfi et al. 1966). Sera containing IgA anti-D as well as IgG anti-D do not as a rule agglutinate red cells suspended in saline although they sensitize red cells to agglutination by anti-IgA. A serum containing potent IgA anti-D, such as a purified IgA fraction from the same serum, which did agglutinate saline-suspended red cells, is mentioned in Chapter 5. In tests on five murine IgA monoclonals, three anti-A and two anti-A,B, agglutinating activity was associated only with tetramers or higher polymers (Guest et al. 1992).
Individual Differences in Response
Individuals vary widely in their response to different antigens. The recognition of nearly all antigens depends on their presentation, bound to HLA class II molecules by antigen-presenting cells, i.e. dendritic cells. Evidently then, specific immune responsiveness is influenced by the products of the HLA-DR alleles (see review by Benacerraf 1981; see also Chapters 6 and 13). Control of antibody responses is also influenced by genes outside the HLA system and segregating independently from it, which control the quantitative production of antibody.
Alterations in Antibody Response in Disease
Subjects with autoimmune disease appear to have an increased risk of forming red cell alloantibodies. One early example was a patient with systemic lupus erythematosus, who formed five different immune alloantibodies (Race and Sanger 1950, p. 240). There have been several reports of a high incidence of alloantibodies in patients with the warm-antibody type of autoimmune haemolytic anaemia (WAIHA) who have been transfused. In three series, the frequency was 32–38% and was as high as 75% in patients who had received more than five transfusions (Branch and Petz 1982; James et al. 1988; reviewed by Garratty and Petz 1994). In these three series, the patient’s serum was absorbed with autologous red cells before being tested for alloantibodies. In another series it was found that 44% of alloantibodies could not be detected before autoabsorption (Wallhermfechtel et al. 1984). There has been one report indicating that red cell alloimmunization is rare in WAIHA (Salama et al. 1992) but the patients’ sera were not absorbed with autologous red cells before being tested and alloantibodies may have been overlooked.
Subjects with hypogammaglobulinaemia have a greatly diminished capacity to form alloantibodies. As described in Chapter 4, their serum may lack anti-A and anti-B. In one case a patient of group A whose serum lacked anti-B was given a series of injections of B substance of animal origin but failed to form anti-B (Barandun et al. 1956).
Among patients with various diseases, receiving regular transfusion over a period of a year or so, only those with chronic lymphocytic leukaemia failed to produce any new red cell alloantibodies; in contrast, patients with acute myeloid leukaemia on intensive chemotherapy produced alloantibodies as frequently as those with aplastic anaemia or gastrointestinal bleeding (Blumberg et al. 1983). In another study of patients receiving regular transfusions, the frequency of formation of red cell alloantibodies was significantly lower in patients with chronic lymphocytic leukaemia than in those with other diagnoses (Blumberg et al. 1984). A case–control study carried out by Bauer et al. (2007) provides evidence that lymphoproliferative disorders in general and symptomatic atherosclerosis are protective against alloimmunization. In contrast, solid malignancy, previous allogeneic peripheral blood progenitor cell transplantation, diabetes mellitus and female sex were identified as risk factors for alloimmunization. Patients on haemodialysis may have a reduced tendency to form red cell alloantibodies; of 405 patients who had received a total of almost 7000 red cell transfusions, only seven developed alloantibodies attributable to the transfusion (Habibi and Lecolier 1983).
In another study, no evidence for a relationship between disease and likelihood of an alloantibody response was found (Higgins and Sloan 2008). These authors consider genetic predisposition to be the primary factor determining alloantibody formation and suggest that the studies described above which correlate particular diseases with risk of alloimmunization are compromised by the small numbers of patients analyzed.
Formation of Immune Red Cell Alloantibodies in Infants
The immune response in newborn infants appears to be similar to that of newborn mice; the response is typically limited to the production of low-titre IgM antibodies, with reduced switching to IgG production and the repertoire of available antibody specificities is highly restricted. In the mouse, the repertoire is 100 times smaller in the newborn than in the adult. In newborn human infants, class II HLA molecules are weakly expressed (Riley 1992) although the response to foreign antigens is normal (Roncarolo et al. 1994). It is uncommon for red cell alloantibodies other than anti-A and -B to be produced in the first few months of life. Some examples are: (1) anti-c in a 7-week-old child of phenotype CCDee, who had been transfused during surgery 6 weeks previously (S Kevy, unpublished observations, reported by Konugres 1978); (2) anti-Lub in a 2-month-old infant who had been transfused 1 month previously (unpublished observations, M. Contreras); (3) IgG anti-E in an infant aged 11 weeks who had received 31 transfusions in the previous 6 weeks, all from donors whose plasma had been screened for alloantibodies (DePalma et al. 1991); and (4) anti-K in a premature infant born at 30 weeks and given 28 red cell transfusions in the first 2 months, anti-K was found for the first time 18 days after the last transfusion; the antibody was still present 1 year later (Maniatis et al. 1993).
In two series of newborn infants transfused with blood from an average of nine donors during the first few months of life no unexpected red cell antibodies were detected. The first series consisted of 53 premature infants, about one-half of whom were tested at least 5 months after birth (Floss et al. 1986) and the second of 90 full-term infants tested not less than 3 weeks after their last transfusion (Ludvigsen et al. 1987). In contrast, in a series of adults, primary immunization to red cell antigens was observed in some 6% of subjects (see below).
Role of the Spleen
In splenectomized subjects, the response to sheep red blood cells (SRBC) is much lower than in control subjects (Rowley 1950; McFadzean and Tsang 1956). However, the spleen is not essential in the formation of antibodies against red cell antigens. For example, the patient described by Collins and colleagues (1950), who formed so many blood group antibodies, had had her spleen removed before receiving the series of blood transfusions that immunized her. Moreover, in subjects with sickle cell disease, whose spleens are usually infarcted and functionless, alloimmunization is no less frequent than in other patients (see later in text).
Association between Weakening of Red Cell Antigens and the Appearance of Allo- or Autoantibodies in the Serum
There are many examples of this phenomenon.
Monoclonal Antibodies
Each kind of antibody molecule is made by a line of plasma cells derived from a single lymphocyte. The progeny of a single cell is called a clone and a monoclonal antibody population consists of the identical molecules produced by such a clone. When an antigen is injected into an animal, numerous different lymphocytes, each producing antibodies with the capacity to react to some extent with the antigen, are stimulated. Polyclonal antibodies consist of such collections of molecules.
In 1975, Köhler and Milstein described a method of obtaining monoclonal antibodies, which depended on fusing mouse lymphocytes with mouse myeloma cells. The lymphocytes were obtained from the spleen of mice immunized with a particular antigen. Unfused splenic lymphocytes die in laboratory culture within 48 h and unfused myeloma cells in the culture die because the composition of growth medium is selected so that it does not support DNA synthesis in these cells. As a result, the fused ‘hybridoma’ cells flourish and cell lines secreting specific antibody that grow indefinitely in culture can be obtained by cloning. Cloning is achieved by culturing the hybrid cells at low cell concentrations in microplates, recovering cells from the well containing the antibody of interest, and then repeating the process two or three times to obtain a stable clone of cells. When stable clones secreting antibody of interest at high concentrations (50–100 µg/ml) are obtained it is possible to culture the cells in large fermenters to obtain grams of antibody for diagnostic or therapeutic use.
The ability to make monoclonal antibodies has proved to be of vast importance, on both a theoretical and a practical level.
Mouse Monoclonal Antibodies
Some monoclonal antibodies produced from lymphocytes from mice injected with human red cells have specificities corresponding to those of human alloantibodies, for example anti-A, -B, -A,B, -H Type 1, -H Type 2, -P, -P1, -Pk, -I, -Y (as in Ley), -Lea, -Leb, -M, -N, -T, -Tn, -k, -Lub (First and Second International Workshop on Monoclonal Antibodies 1987, 1990) and -Ge3 (Loirat et al. 1992). Others react with structures carrying the antigenic determinants rather than the antigens themselves. For example, they may react with glycophorin A, rather than with M or N; or they may react with Rh-related glycoprotein and thus with all human red cells except those that are Rhnull; or they may react with Kell glycoprotein and thus with all except KO cells (see Chapter 6). Murine monoclonal antibodies against A and B and against complement components have largely replaced polyclonal reagents in diagnostic blood typing procedures.
Human Monoclonal Antibodies
The first human monoclonal antibodies with blood group specificity were produced by transformation of human B lymphocytes with Epstein–Barr virus (EBV). The lymphoblastoid cells that result have the property of growing in tissue culture and of secreting antibody. By taking lymphocytes from Rh D-immunized donors, both IgG (Koskimies 1980) and IgM (Boylston et al. 1980) anti-D have been obtained. EBV-transformed cell lines are unstable, often ceasing to produce antibody after a few months (Melamed et al. 1985), although many stable EBV-transformed cell lines have been described (Goosens et al. 1987; Kumpel et al. 1989a). Another way of obtaining stable cell lines is to fuse human lymphocytes with murine myelomas to form heteromyelomas (see review by Thompson and Hughes-Jones 1990).
Examples of monoclonal anti-D have been used in analysing the different D epitopes (see Chapter 5) and in the experimental clearance of D-positive red cells from the circulation of volunteers (see Chapter 10); they are used routinely in blood grouping (see Chapter 8). Other human monoclonal antibodies available as diagnostic reagents include anti-C, -c, -E, -e, -G, -K, -Jka, -Jkb, -H, -Lea, -Leb, -IgG and -C3d.
Some Disadvantages of Monoclonal Antibodies
As mentioned elsewhere in this chapter, changes in pH alter the binding affinity of antibodies. The effect is particularly striking with monoclonal antibodies and may have a profound effect on apparent specificity in tests in a system such as HLA, which involves many similar but non-identical antigens. Monoclonal antibodies which give non-specific reactions at normal pH can be made monospecific by lowering pH to 6.0 (Mosmann et al. 1980).
A problem associated with some high-affinity monoclonal antibodies is crossreactivity. It may seem paradoxical that an antibody apparently specific for a single antigenic determinant should crossreact at all. Nevertheless, single antibody molecules are not specific for one particular epitope. The combining site on the end of the Fab arm is capable of binding closely with a number of different epitopic configurations (Talmage 1959). The higher the affinity of an antibody, the more it tends to crossreact (Steiner and Eisen 1967). Presumably, the more strongly an antibody combines with any particular determinant, the more certain it is to bind to some extent to related antigenic determinants. By no means all monoclonal antibodies have a high affinity. On the contrary, the affinities of monoclonal antibodies produced by different clones are similar to those of polyclonal antibodies, although all antibodies of a single clone have the same affinity, whereas the antibodies in a polyclonal population have a range of affinities. Monoclonal antibodies even of average affinity are more likely than polyclonal antibodies to crossreact. This is because all the molecules of a monoclonal antibody population have the same paratope and will therefore all recognize the same crossreacting antigen. In contrast, in a population of polyclonal antibodies, the paratopes will differ and only a proportion of the molecules will recognize any particular crossreacting antigen.
Crossreactivity explains the demonstration that certain examples of monoclonal anti-D react with the cytoplasmic component vimentin (Thorpe 1989, 1990), giving rise to the false conclusion that D is present on tissue cells. On the other hand, the reaction of certain monoclonal anti-A with B cells and of certain monoclonal anti-B with A cells is due to the potency of the antibodies concerned which enables them to detect the small amounts of B and A, respectively produced by A and B subjects (see Chapter 4).
A different problem is provided by monoclonal antibodies with ‘pseudo-specificity’, for example: (1) an anti-glycophorin B, which, in agglutination tests, behaved as anti-S but in other tests reacted with both s+ and S+ red cells (Green et al. 1990) and (2) an anti-J chain that reacted more strongly with Gm(a+) than with Gm(a−) molecules but could be shown to be reacting with the same determinant on the γ chain in both cases (De Lange 1988).
Copying Variable Regions and Constructing New Antibodies
For the construction of new antibodies, the genes encoding the V regions can be amplified using the PCR and can then be cloned into expression vectors (e.g. bacteriophage). Complete antibody V domains with specificity identical to the parent antibody can then be displayed on the surface of the bacteriophage. Completely new antibodies can be constructed (McCafferty et al. 1990). Another approach has been to link VH and VL gene repertoires together at random to encode a repertoire of single-chain FV (scFV) antibody fragments, which are then displayed on the surface of bacteriophage. Phage are selected by binding to the surface of mobilized antigen (red cells) and then used to infect E. coli. Antibodies produced include anti-B, -D, -HI, -E and -Kpb (Marks et al. 1993) and -HPA-1a (Griffin and Ouwehand 1995).
Frequency of Immune Red Cell Alloantibodies
By definition, an immune red cell alloantibody is one that develops in a subject who has been exposed to a red cell alloantigen. Evidently then, the frequency of immune red cell antibodies will be zero in subjects who have never been transfused or been pregnant, and will be greatest in subjects who have received many transfusions. For two reasons, pregnancies constitute a smaller stimulus than transfusions: first, the number of foreign antigens is limited to those possessed by the father of the fetus (although, of course, some women have infants by more than one man), and, second, in many pregnancies the amount of red cells transferred from fetus to mother is too small to stimulate a primary response.
The frequency of alloantibodies is expected to be relatively low in blood donors compared with patients in hospital requiring blood transfusion; blood donors have a lower average age and are far less likely to have received blood transfusions in the past. The frequency with which particular antibodies are found depends on the sensitivity of testing (usually relatively low in screening healthy donors) and on the ethnic group being tested (e.g. anti-D is rare in Chinese people).
D is the most immunogenic red cell antigen (excepting A and B), but the frequency of immunization to D has been reduced to a low level, first, by using only D-negative donors for transfusion to D-negative subjects and, second, by administering anti-D Ig to D-negative women who would otherwise become immunized as a result of a pregnancy with a D-positive infant. As a result of these measures, the frequency with which anti-D is encountered has fallen greatly in the last few decades.
In contrast with the diminishing frequency of anti-D, that of anti-K, -Fya, etc. has increased, evidently due to the increased number of multitransfused patients and the absence of measures for preventing immunization to antigens other than D. A relatively small percentage (ca.10%) of patients make alloantibodies upon transfusion but reliable methods for identifying such individuals are lacking. There is some evidence suggesting that the presence of inflammation in a patient increases the likelihood of alloantibody production. In a study of 193 patients with inflammatory bowel disease and a history of transfusion or pregnancy a 2.5 fold increase in red cell antibody prevalence was found compared with controls. Patients with inflammatory bowel disease had higher C-reactive protein levels during transfusion than controls (Papay et al. 2012). Genetic factors also predispose to alloimmunization. The rs660 polymorphism in Ro52 has been identified as a marker of relevance to alloimmunization in sickle cell disease (Tatari-Calderone et al. 2009).
Frequency of Immune Anti-D (Including Anti-DC and Anti-DE)
In Healthy Blood Donors
In more than 200 000 donors screened between 1971 and 1975 in Seattle, the frequency was 0.22% (Giblett 1977). In 36 000 donors screened in London in 1988, the frequency (0.25%) was almost identical although in 35 000 screened in 1990 the frequency was 0.16% (R Knight, personal communication).
In Pregnant Women
Before the introduction of immunoprophylaxis, anti-D was found in approximately 1 in 170 pregnant white women, both in England (Walker 1958) and in North America (Walker 1984). Following the introduction of immunoprophylaxis with anti-D in the late 1960s, the frequency with which anti-D is found has fallen progressively and was, for example, 1 in 963 in 1988 in one North American survey (RH Walker, personal communication 1991) and 1 in 1600 in another (Heddle et al. 1993). The frequency in one English region for 1988 had fallen to a lesser extent, being 1 in 497 (GJ Dovey, personal communication), although a slightly greater fall, namely to one in 584, was found in another English region for 1993–95 (A Rankin, personal communication). Figures for the changing incidence of HDN are given in Chapter 12.
In Pre-Transfusion Tests on Potential Recipients
Although there appears to have been a fall between the mid-1950s and mid-1970s in the frequency with which anti-D (and -DC) was found in transfusion recipients, there has been no obvious fall since then. Some figures are as follows: in 1956–57, 0.77%; in 1974–75, 0.52%, based on testing 60 000 patients in Seattle (Giblett 1977); in 1970–75, 0.55%, in 1976–81, 0.29% and in 1982–87, 0.27%, based on screening about 100 000 patients in each 5-year period in Michigan (Walker et al. 1989). In a smaller series (more than 12 000 patients) tested in England in 1990, 0.56% (J Sangster, personal communication).
In Recipients Following Solid Organ Transplants
The frequency with which anti-D develops in D-negative recipients of D-positive grafts and the apparent influence of cytotoxic drugs is mentioned in Chapter 5.
Frequency of Immune Red Cell Antibodies Other Than Anti-D
In Healthy Blood Donors
In the two series referred to above, the frequencies were 0.10% in Seattle in 1975 (Giblett 1977) and in London 0.19% in 1988 and 0.18% in 1990 (unpublished observations, M Contreras). In the London series, about 30% of the antibodies were anti-E and 20% were anti-K.
In Pregnant Women
In approximately 175 000 women (about 85% of whom were D positive), antibodies other than anti-D were found in 0.14%; rather more than one-half of these were within the Rh system and, of these, one-half were anti-E; the next commonest antibody, found in 0.025% of the women, was anti-K (Kornstad 1983). In a more recent survey, clinically significant antibodies other than anti-D were found in 100 out of 17 568 women, but in 58 instances the antibody was present in the first trimester and it was concluded that the frequency of antibodies stimulated by the current pregnancy was 42 out 17 568 or approximately 0.24% (Heddle et al. 1993).
In Pre-Transfusion Tests on Potential Recipients
Frequencies were: in 1974–75, 1.12% (Giblett 1977); in 1970–75, 0.39%; in 1976–81, 0.45% and in 1982–87, 0.6% (Walker et al. 1989).
In Random Recipients Following Transfusion
The figures in the preceding paragraph are for patients, only some of whom had been transfused or been pregnant previously. In a prospective study of 452 patients, all of whom had been transfused in relation to elective surgery, pre-transfusion samples and 10 serial, 2-weekly, post-transfusion samples were taken. Additional red cell alloantibodies developed in 38 out of 452 (8.2%) patients between 2 and 18 weeks after transfusion, but 10 of these had been transfused before and one had been pregnant, leaving an apparent frequency of primary immunization of about 27 out of 441 (6.1%); 13 out of the 27 antibodies were anti-E and if these are excluded (because their immune nature is in some doubt) the frequency falls to 14 out of 428 (3.3%). Of all the newly found antibodies, 50% were initially detectable only by a two-stage papain technique or by the manual polybrene test, and not by the indirect antiglobulin test (IAT) (Redman et al. 1996).
Relative Frequencies of Antibodies Other Than Anti-D
Combined figures from 20 different blood grouping laboratories were reported by Grove-Rasmussen (1964); these figures and those from a much smaller series reported by Tovey (1974) are shown in Table 3.6. No information was given as to the relative numbers of parous women and transfusion recipients in the two series. As will be seen, Rh antibodies (other than anti-D) constituted about 54% of the total and anti-K and -Fya about 40%, leaving only 5% for all other specificities. In the series of Walker and colleagues (1989), if the figures for the three 5-year periods (in each of which approximately 100 000 patients were screened) are pooled, the absolute frequencies for antibodies of various specificities were as follows: Rh antibodies other than anti-D, 0.22%; anti-K, 0.19%; anti-Fya, 0.05%; and anti-Jka, 0.035%.
Table 3.6 Relative frequency of immune red cell antibodies* (excluding anti-D, -CD and -DE): (a) and (b) in transfusion recipients (and some pregnant women), (c) associated with immediate haemolytic transfusion reactions and (d) associated with delayed haemolytic transfusion reactions.
Patients with Thalassaemia
These patients are usually transfused about once per month, starting in the first few years of life. In some series in which patients have been transfused with blood selected only for ABO and D compatibility, antibodies, mainly of Rh or K specificities, have been found in more than 20% of patients. For example, out of 973 thalassaemics transfused with an average of 18 units per year from about the age of 3 years, 21.1% had formed clinically significant antibodies after about 6 years; 84% of the antibodies were within the Rh or K systems; about half the immunized patients made antibodies of more than one specificity. Of 162 patients transfused from the outset with red cells matched for Rh and K antigens, only 3.7% formed alloantibodies compared with 15.7% of 83 patients of similar age, transfused with blood matched only for D (Spanos et al. 1990). Chinese patients are less likely to have anti-K but more prone to develop antibodies against Miltenberger antigens (Cheng et al. 2012).
The incidence of antibody formation is less when transfusion is started in the first year of life (Economidou et al. 1971). The induction of immunological tolerance by starting repeated transfusions at this time was believed to account for the low rate of alloimmunization, namely 5.2%, observed in a series of 1435 patients (Sirchia et al. 1985).
Alloimmunization in Sickle Cell Disease
In a survey of 1814 patients from many centres, the overall rate of alloimmunization was 18.6%. The rate increased with the number of transfusions and, although alloimmunization usually occurred with less than 15 transfusions, the rate continued to increase as more transfusions were given. The commonest specificities were anti-C, -E and -K; 55% of immunized subjects made antibodies with more than one specificity (Rosse et al. 1990). In another series, the incidence of alloimmunization was somewhat higher; out of 107 patients who received a total of 2100 units, 32 (30%) became immunized and 17 of these formed multiple antibodies; 82% of the specificities were anti-K, -E, -C or -Jkb. Those patients who formed antibodies had had an average of 23 transfusions; those who did not had had an average of 13; 75% of antibodies had developed by the time of the 21st transfusion (Vichinsky et al. 1990).
The finding that the percentage of patients forming antibodies increases with the number of transfusions has been documented in previous series (Orlina et al. 1978; Reisner et al. 1987). In the latter series, 50% of patients who had received 100 or more transfusions had formed antibodies. Lower alloimmunization rates have been reported in paediatric sickle cell patients receiving chronic erythrocytapheresis compared to single transfusions (Wahl et al. 2012). Erythrocytapheresis is the method of choice for sickle cell patients. It is more effective in lowering the HbS level and preventing iron overload.
It has been suggested that in sickle cell disease the rate of alloimmunization is due partly to racial differences between donors (predominantly white people) and patients (black people). The antibodies formed most commonly are anti-K, -C, -E and -Jkb, and the frequencies of each of the corresponding antigens are significantly higher in white people than in black people (Vichinsky et al. 1990). It has been pointed out that when one considers the probability of giving at least one incompatible unit when 10 units are transfused, the differences for C, E and Jkb between white and black donors become very small, only that for K remaining substantial, namely 0.178 with black donors and 0.597 with white (Pereira et al. 1990), so that the use of white donors for black donors may not play a large role in inducing the formation of red cell alloantibodies. In any case, the conclusion is that for patients with sickle cell disease, as for those with thalassaemia, it is worth giving blood matched for Rh antigens and for K. This conclusion is implied by the findings of Rosse and co-workers (1990) and was reached earlier by Davies co-workers (1986). These latter authors found that two of their patients, both of the phenotype Dccee, which is much commoner in black people than in white people, had developed anti-C and anti-E, and they recommended that Dccee patients with sickle cell disease should be given C-negative, E-negative blood. The availability of DNA-based methods for blood group genotyping makes it feasible to screen large donor inventories to find blood compatible at more blood group loci than Rh and K, so further reducing the chances of alloimmunization in sickle cell patients (reviewed by Anstee 2009; see also Chapter 8).
Alloimmunization Following Solid Organ Transplants
Out of 704 recipients of transplants of heart, lung or both, who were followed up, new alloantibodies appeared, usually only transiently in 2.1%. The frequency with which anti-D was formed is mentioned in Chapter 5; the commonest other specificities were anti-E and anti-K. The low incidence was attributed to immunosuppressive therapy (Cummins et al. 1995).
Relative Importance of Different Alloantibodies in Transfusion
As discussed in Chapter 11, anti-A and anti-B must be regarded as overwhelmingly the most important red cell alloantibodies in blood transfusion because they are most commonly implicated in fatal haemolytic transfusion reactions. Rh antibodies are the next most important mainly because they are commoner than other immune red cell alloantibodies. For example, in the series of Grove-Rasmussen and Huggins (1973), out of 177 antibodies associated with haemolytic transfusion reactions (omitting 30 cases in which anti-A and anti-B were responsible and also omitting cases in which only cold agglutinins were found, which were unlikely to have been responsible for red cell destruction), 95, including 35 examples of anti-D, were within the Rh system. Estimates of frequencies with which other red cell alloantibodies were involved in immediate and delayed haemolytic transfusion reactions are shown in Table 3.6.
The figures given in Table 3.6 show that the frequencies with which the different red cell alloantibodies were involved in immediate haemolytic transfusion reactions were similar to the frequencies with which the same red cell alloantibodies were found in transfusion recipients. On the other hand, the figures for delayed haemolytic transfusion reactions show one very striking difference in that antibodies of the Jk system were very much more commonly involved than expected from a frequency of these antibodies in random transfusion recipients. Possibly this discrepancy is due to the fact that red cell destruction by Kidd antibodies tends to be severe so that perhaps delayed haemolytic reactions are more readily diagnosed when these antibodies are involved, or, to put it in another way, delayed haemolytic transfusion reactions associated with other red cell alloantibodies may tend to be missed.
Perhaps a more important reason why Kidd antibodies tend to be relatively frequently involved in delayed haemolytic transfusion reactions may be that they are difficult to detect, particularly when present in low concentration. Moreover, unlike some antibodies, for example anti-D, which after having become detectable remain detectable for long periods of time, Kidd antibodies tend to disappear (see Chapter 6).
Although examples of anti-Lea and some examples of anti-Leb are active at 37°C in vitro, they have very seldom been the cause of haemolytic transfusion reactions, mainly because Lewis antibodies are readily neutralized by Lewis substances which are present in the plasma of the transfused blood.
Although most antibodies that are active at 37°C in vitro are capable of causing red cell destruction, there are exceptions (see Chapter 11; reviewed by Poole and Daniels 2007). In some cases the explanation may lie in the IgG subclass of the antibody and in others, perhaps, in the paucity of antigen sites.
Cold alloantibodies such as anti-A1, anti-HI, anti-P1, anti-M and anti-N are usually inactive in vitro at 37°C and are then incapable of bringing about red cell destruction. Occasional examples which are dubiously active at 37°C but active at 30°C or higher may bring about the destruction of small volumes of incompatible red cells given for the purpose of investigation. References to very rare examples of anti-A1 and anti-P1, anti-M and anti-N that have caused haemolytic transfusion reactions will be found in later chapters.
Relative Potency (Immunogenicity) of Different Antigens
An estimate of the relative potency of different red cell alloantigens can be obtained by comparing the actual frequency with which particular alloantibodies are encountered with the calculated frequency of the opportunity for immunization (Giblett 1961). For example, suppose that in transfusion recipients anti-K is found about 2.5 times more commonly than anti-Fya (see Table 3.6a and b). The relative opportunities for immunization to K and Fya can be estimated simply by comparing the frequency of the combination K-positive donor, K-negative recipient, i.e. 0.09 × 0.91 = 0.08, with the frequency of the combination Fy(a+) donor, Fy(a−) recipient, i.e. 0.66 × 0.34 = 0.22. Thus the opportunity for immunization to K is about 3.5 times less than that for Fya (0.08 vs. 0.22). In summary, although opportunities for immunization to K are 3.5 times less frequent than those to Fya, anti-K is in fact found 2.5 times more commonly than anti-Fya, so that overall, K is about nine times more potent than Fya. If a single transfusion of K-positive blood to a K-negative subject induces the formation of serologically detectable anti-K in 10% of cases (see Chapter 6) it is, therefore, predicted that the transfusion of a single unit of Fy(a+) blood to an Fy(a−) subject would induce the formation of serologically detectable anti-Fya in about 1% of cases.
Using earlier data, Giblett (1961) calculated that c and E were about three times less potent than K, that Fya was about 25 times less potent and Jka 50–100 times less potent. Tormey and Stack (2009) calculated the relative immunogenicity of different blood group antigens in males to avoid influence of pregnancy. They also took account of antibody persistence and excluded antibodies reactive only at room temperature on the assumption that such antibodies were naturally occurring. Their results indicate that the antigens Jka, Lua and Cw are significantly more immunogenic than suggested using the Giblett method (4.81, 4.26 and 3.68 fold greater respectively). In contrast the immunogenicity of C,c,Fya and S decreased slightly.
Transfusion and Pregnancy Compared As a Stimulus
In considering the risks of immunization by particular red cell alloantigens, the effect of transfusing multiple units of blood and the relative risks of immunization by transfusion and pregnancy must be discussed.
When an antigen has a low frequency, for example K, with a frequency of 0.09, the chance of receiving a unit containing the antigen increases directly with the number of the units transfused, up to a certain number (11 in this instance). On the other hand, when an antigen has a high frequency, for example c, frequency 0.8, the chance of exposure is high with only a single unit and increases only slightly as the number of units transfused increases. The point can be illustrated by calculating an example. For the transfusion of a single unit, the chance that the donor will be K positive and the recipient K negative is 0.09 × 0.91 = 0.08; the corresponding risk of incompatibility from c is 0.8 × 0.2 = 0.16; the relative risk from the two antigens (K/c) is thus 0.5:1.0. When 4 units are transfused, the chance of K incompatibility (at least one donor K positive and the recipient K negative) is 0.31 × 0.91 = 0.28 and of c incompatibility 0.997 × 0.2 = approximately 0.2, so that the relative risk (K/c) is now 0.28:0.2 or 1.4:1 (Allen and Warshaw 1962). To summarize, the relative risk of exposure to K compared with c is about three times as great with a 4-unit blood transfusion as with a 1-unit transfusion.
When the antigen has a low frequency, opportunities for making the corresponding antibody are much lower from pregnancy than from blood transfusion, assuming that a woman has only one partner and that in transfusion many different donors are often involved. For example, in women who have three pregnancies the chance that in two of them the fetus will be c incompatible with its mother is about three times greater than that two of them will be K incompatible (Allen and Warshaw 1962).
These theoretical considerations are supported by actual findings: among women sensitized by blood transfusion alone, anti-K was almost three times more common than anti-c (32:12), whereas among women sensitized by pregnancy alone the incidence of the two antibodies was similar (9:7) (Allen and Warshaw 1962).
When a woman carries a fetus with an incompatible antigen, she is far less likely to form alloantibodies than when she is transfused with blood carrying the same antigen. Presumably the main reason for the difference is simply that in many pregnancies the size of transplacental haemorrhage does not constitute an adequate stimulus for primary immunization.
In two different series in which anti-c was detected in pregnant women there was a history of a previous blood transfusion in over one-third of the women (Fraser and Tovey 1976; Astrup and Kornstad 1977).
The Effect of Rh D Immunization on the Formation of Other Red Cell Alloantibodies
Among Rh D-negative volunteers deliberately injected with D-positive red cells, those who form anti-D tend also to form alloantibodies outside the Rh system, whereas those who do not form anti-D seldom form any alloantibodies at all. In one series of 73 subjects who formed anti-D, six formed anti-Fya, four formed anti-Jka and four formed other antibodies; by contrast, amongst 48 subjects who failed to form anti-D, not one made any detectable alloantibodies (Archer et al. 1969).
An association between the formation of anti-D and that of antibodies outside the Rh system was previously noted by Issitt (1965) in women who had borne children.
Several series in which D-negative subjects have been deliberately immunized with D-positive red cells are available for analysis. In some series, donors and recipients were tested for other red cell antigens so that the numbers at risk from these other antigens are known. In other series, donors and recipients were not tested, or only donors were tested, for antigens other than D, so that it is only possible to estimate the numbers at risk from the known incidence of the relevant antigens in a random population. In Table 3.7 estimates of the immunogenicity of K, Fya, Jka and s in three circumstances are listed: (1) in subjects receiving D-compatible red cells; (2) in D-negative recipients receiving D-positive red cells but not making anti-D; and (3) in D-negative recipients receiving D-positive red cells and making anti-D.
Table 3.7 Response to K, Fya, Jka and s in relation to RhD compatibility of injected red cells.
The data summarized in Table 3.7 emphasize the tremendously increased response to antigens outside the Rh system in subjects responding to D. In subjects who formed anti-D and had the opportunity of making other antibodies, 50% formed anti-K. The incidence of anti-Fya, anti-Jka and anti-s in those who could respond was about 20% in each instance. In deliberately immunizing Rh D-negative subjects to obtain anti-D, it is clearly very important to choose donors who cannot stimulate the formation of antibodies such as anti-K, -Fya or -Jka.
The question arises whether non-responders to D are also non-responders to other red cell antigens. The data shown in Table 3.7 do not answer the question, as although no alloantibodies were formed by non-responders to D, only two such antibodies were made by recipients of D-compatible red cells, and much larger numbers are needed to discover whether there is any difference between the two categories. However, a retrospective study examining the formation of antibodies to Fy, Jk, and MNS system antigens alone or in combination with anti-E and/or anti-K demonstrated no enhancing effect of antibody formation to antigens E and/or K on the immune response to antigens Fy, Jk and/or MNS, a conclusion consistent with propensity to form alloantibodies of any specificity being dependent on the responder status of the transfusion recipient (Schonewille and Brand 2008). Higgins and Sloan (2008) provide persuasive evidence for the existence of a small population of individuals (13%) having a genetic predisposition to make alloantibodies (responders) and make the salient point that the use of DNA-based typing for extended blood group genotype determination should be used selectively to prevent alloimmunization in this responder population. The ability of responders to produce anti-human glycophorin A in a mouse transfusion model was related to reduced suppressor activity of T regulatory cells. Furthermore, responders developed additional alloantibodies whereas non-responders were resistant to alloimmunization in this model (Bao et al. 2009).
Competition of Antigens
In considering the possible interference of immunization to one red cell antigen on the response to another, the fact that both antigens may be carried on the same red cells must be taken into account. As soon as antibody has been formed to one antigen it will tend to bring about rapid destruction of the red cells and this process may interfere with the immune response to a second antigen.
There is quite extensive evidence that red cells carrying two antigens, for the first of which there is a corresponding antibody in the subject’s serum, may fail to immunize against the second antigen. The best known example is the protective effect against Rh D immunization exercised by ABO incompatibility (see Chapter 5). ABO incompatibility has also been shown to protect against immunization to c (Levine 1958), K (Levine, quoted by Race and Sanger 1968, p. 283), and a number of other antigens including Fya, Jka and Dia (Stern 1975). The effect of passively administered anti-K on the response to D carried on D-positive, K-positive red cells is described in ‘Suppression of the immune response by passive antibody’.
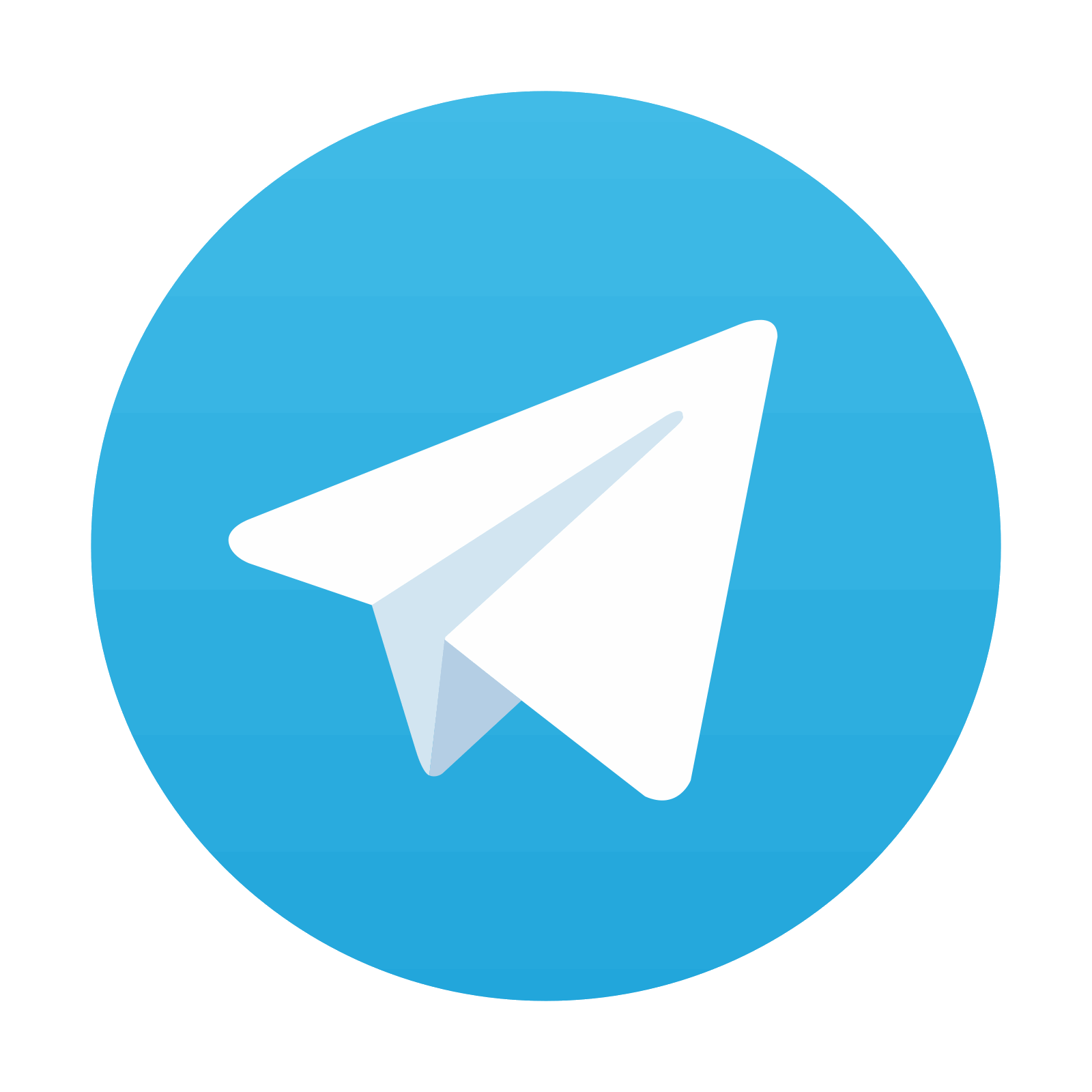
Stay updated, free articles. Join our Telegram channel
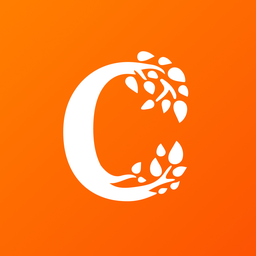
Full access? Get Clinical Tree
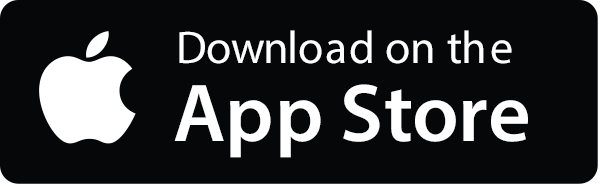
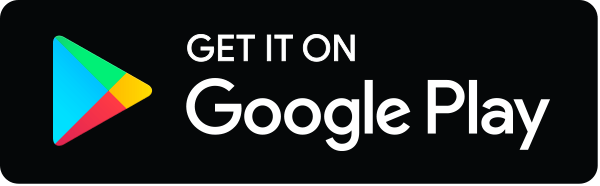