Fig. 12.1
Annexin A1-mediated homing of dendritic cells. Annexin A1 (ANXA1) is released from cancer cells in response to certain therapeutic approaches including the anthracycline- and oxaliplatin-based chemotherapeutic induction of immunogenic cell death. Driven by chemotaxis, immature dendritic cells (iDCs) are homed in on their target in a FPR1-dependent fashion, finally leading to a close proximity of dying cancer cells and antigen-presenting cells. FPR1, formyl peptide receptor 1
The aforementioned findings, which have been obtained in mice, are supported by epidemiological studies in cancer patients. A loss-of-function mutation in FPR1 (A299G), which affects the intracellular domain of the protein within its N-terminus abolishing the dimerization of the receptor required for its activation [35], had negative prognostic features in two types of cancer. Breast cancer patients bearing one loss-of-function allele of FPR1 exhibited a shorter progression-free and overall survival upon adjuvant anthracycline-based chemotherapy than patients bearing two normal alleles of FPR1. This finding was obtained for two independent cohorts of breast cancer patients [32]. Moreover, colorectal cancer patients bearing two loss-of-function alleles of FPR1 had a statistically shorter survival upon adjuvant oxaliplatin-based chemotherapy than patients bearing one or two normal alleles of FPR1 [32]. The mechanistic bases for these differences are not understood yet. In addition, it appears that mammary carcinoma cells express lower ANXA1 levels than their normal epithelial counterparts [32], perhaps reflecting immunoselection in favor of cancers that lack the DAMP ANXA1.
12.3 ATP
In response to treatment with chemotherapeutics in vitro, cancer cells release adenosine triphosphate (ATP) into the culture supernatant, an event that can be visualized by a reduction in quinacrine-labeled, ATP-containing lysosomal compartments [36]. The accompanying increase in extracellular levels of ATP can be measured by means of a firefly luciferase construct that is tethered to the cancer cell surface and that detects pericellular ATP upon addition of d-luciferin [37, 38]. This latter system is suitable for measuring extracellular ATP in vivo in tumor-bearing mice, in which the luminescence signal strongly increases 2 days post-chemotherapy [25]. The mechanism of ATP release has not been entirely elucidated yet appears to involve a lysosomal secretion mechanism that depends on at least two processes, namely, a premortem autophagy response and caspase activation. Autophagy must occur to allow ATP to redistribute from lysosomes to autolysosomes and to be secreted by a mechanism that requires the lysosomal-associated membrane protein 1 (LAMP1), which translocates to the plasma membrane in a caspase-dependent manner. The release of ATP additionally involves the caspase-mediated activation of the Rho-associated coiled-coil-containing protein kinase (ROCK1) resulting in myosin II-dependent membrane blebbing as well as the opening of pannexin 1 (PANX1) channels, subsequent to their cleavage by caspases. While autophagy and LAMP1 do not affect PANX1 channel opening, PANX1 is required for the ICD-associated translocation of LAMP1 to the plasma membrane [39]. Hence, apoptosis-associated ATP release is a complex process that is abolished in autophagy-deficient tumors, knowing that inactivation of autophagy occurs rather frequently, especially during early oncogenesis [25, 40–43]. Necroptotic signaling via RIPK3 and the mixed lineage kinase domain-like (MLKL) pseudokinase may also contribute to ATP release [18], although it is not known whether this process also requires premortem autophagy to be induced. In any case, it appears that cancer cells manipulated to suppress the autophagic process fail to undergo ICD and do not reduce their growth upon treatment with anthracyclines or oxaliplatin in vivo [25]. A similar abolition of ICD and chemotherapeutic responses can be obtained by overexpressing the ectonucleoside triphosphate diphosphohydrolase 1 (ENTPD1, also known as the ectoATPase CD39) on the cancer cells [25, 44].
Extracellular ATP acts on two classes of purinergic receptors, namely, the metabotropic P2Y2 and the ionotropic P2X7 receptors. P2Y2 receptors facilitate the ATP-mediated chemotaxis of myeloid cells (dendritic cell precursors, neutrophils and macrophages) into the tumor bed post-chemotherapy (Fig. 12.2). Both autophagy-deficient and CD39-overexpressing cancers fail to accumulate myeloid cells post-chemotherapy in the tumor bed [25], and a similar effect can be obtained upon pharmacological inhibition of P2Y2 [44]. P2X7 receptors facilitate the ATP-stimulated activation of the NLR family pyrin domain containing 3 (NLRP3) inflammasome in dendritic cells, which then triggers the secretion of interleukin-1β (IL1β) and the IL1β-dependent priming of tumor antigen-specific CD8+ T cells [45]. Indeed, neutralization of P2Y2 or IL1 receptors and knockout of P2X7, NLRP3, or caspase-1 abolish the capacity of the immune system to mount a protective immune response against cancer cells that succumb to ICD [45].


Fig. 12.2
ATP-dependent recruitment and activation of dendritic cells. The autophagy-dependent lysosomal secretion of ATP from cancer cells that undergo immunogenic cell death leads to the recruitment and activation of immature dendritic cells (iDCs). Extracellular ATP acts on purinergic receptors of the metabotropic P2Y2 and the ionotropic P2X7 type. Most prominently P2Y2 receptors drive the ATP-mediated chemotaxis of myeloid cells including immature dendritic cells (iDCs) into the tumor bed post-chemotherapy. In summary, ATP release from the dying cancer cells leads to an enrichment of the tumor bed with immune cells. P2RX7, purinergic receptor P2X7; P2RY2, purinergic receptor P2Y2
The aforementioned interaction between extracellular ATP and purinergic receptors again appears clinically relevant. Indeed, in breast cancer patients treated with adjuvant chemotherapy, the absence of autophagy has a negative impact on the local immune response with an unfavorable ratio of CD8+ T lymphocytes over forkhead box P3+ (FOXP3+) regulatory T cells. Such observation correlates with poor patient survival [46]. Similarly, high expression of ATP-degrading ectoenzymes such as CD39 and the ecto-5′ nucleotidase NT5E (best known as CD73) indicates poor prognosis in multiple distinct cancers including breast and ovarian cancers [47, 48]. Finally, a loss-of-function mutation in P2X7 has been linked to poor prognosis in a segment of breast cancer patients that are treated with anthracycline-based adjuvant chemotherapy [45].
Experimentally, it is possible to stimulate autophagy, ATP release, and consequent myeloid cell recruitment and anticancer immune responses by fasting or by non-immunosuppressive autophagy inducers that fall into the class of “caloric restriction mimetics” (CRMs) [49–52]. Several CRMs including hydroxycitrate can be used in mouse models to improve anticancer immunosurveillance and to boost the anticancer immune responses elicited by ICD-inducing chemotherapeutics [49]. Whether this strategy is applicable to cancer patients awaits urgent clarification.
12.4 Calreticulin
Calreticulin (CALR) is the most abundant protein in the lumen of the endoplasmic reticulum (ER). In the context of ICD, a fraction of CALR translocates to the surface of the plasma membrane (and it is possible that another fraction of CALR is secreted as well) [23, 26, 53, 54]. The complex mechanisms that underlie CALR exposure are linked to the apical phosphorylation of eukaryotic initiation factor-2α (eIF2α) in the context of an ER stress response that culminates in the activation of an eIF2α kinase (EIF2K) such as EIF2K2 (best known as PKR) and EIF2K3 (best known as PERK) and/or in the inhibition of the corresponding phosphatase (composed by the catalytic subunit PP1 and the regulatory subunit GADD34) [26, 55, 56]. Downstream of eIF2α phosphorylation, caspases (and in particular caspase-8, CASP8) are activated, and calreticulin is transported to the cell surface following anterograde ER-Golgi traffic and soluble N-ethylmaleimide-sensitive factor attachment receptors (SNARE)-dependent exocytosis that involves the vesicle-associated membrane protein 1 (VAMP1) and the synaptosomal-associated protein 23 (SNAP23) [55].
Once on the cell surface, CALR acts as an “eat-me” signal to facilitate the transfer of tumor-associated antigens to dendritic cells [57], which express the CALR receptor low-density lipoprotein receptor-related protein 1 (LRP1, best known as CD91; Fig. 12.3) [58]. CALR can be locally antagonized by CD47, which is constitutively expressed on cancer cells and can function as a “don’t eat me” signal [59]. Knockdown of PERK, CASP8, CALR, or SNAP23 as well as pharmacological inhibition of caspases and anterograde ER-Golgi transport is sufficient to abolish ICD in vitro and in vivo [55]. Conversely, stimulation of eIF2α phosphorylation by thapsigargin (which activates PERK) or inhibitors of PP1 can stimulate CALR exposure and enhance anticancer immune responses in vivo, in the context of chemotherapy [55, 60]. Coating of cancer cells that are deficient in the CALR exposure pathway with recombinant CALR protein (which binds to the plasma membrane surface, presumably via interaction between its lectin domain and the glycocalyx) can restore deficient ICD [26, 61]. Similarly, intratumoral injection of CALR can enhance the chemotherapy-elicited immune response and improve tumor growth inhibition in vivo, in mouse models [62].


Fig. 12.3
Calreticulin as de novo uptake signal for dendritic cells. The endoplasmic reticulum (ER) stress-mediated exposure (or release) of the ER chaperone calreticulin (CALR) to the surface of the plasma membrane in the course of immunogenic cell death (ICD) serves as de novo uptake signal for dendritic cells. The binding of CALR to the low-density lipoprotein receptor-related protein 1 (LRP1) receptor expressed on dendritic cells (DC) serves the transfer of tumor-associated antigens to DC
There is widespread evidence that CALR expression and exposure contribute to anticancer immunosurveillance in vivo, in cancer patients. Low intracellular CALR expression levels have been correlated with a low presence of CALR on the cell surface, both in acute myeloid leukemia (AML) and in non-small cell lung cancer (NSCLC), as well as reduced phosphorylation of eIF2α [59, 63, 64]. In AML, reduced expression of CALR protein has a negative impact on progression-free and overall survival post-chemotherapy, correlating with poor T cell-mediated immune responses against AML-associated tumor antigens [59, 64]. In NSCLC, approximately 15% of the patients have barely detectable CALR protein in cancer cells, correlating with dismal prognosis, reduced infiltration by DC-LAMP+ dendritic cells and CD8+ T lymphocytes [63]. Of note, low CALR expression supersedes in importance the TNM classification of NSCLC with respect to prognosis, meaning that patients with CALRlow stage 1 NSCLC exhibit a poorer survival than stage 3 and stage 4 patients bearing CALRhigh cancers [63]. These results have been confirmed for two distinct NSCLC cohorts by detecting CALR protein with immunohistochemistry [64], as well as for an additional NSCLC cohort by measuring CALR mRNA levels and its correlations with metagenes reflecting the presence of CTL and dendritic cells [65]. Similarly, in ovarian cancer, high levels of CALR mRNA expression have a favorable impact on patient survival, if combined with the analysis of activated dendritic cells [65]. Conversely, high CD47 expression has a negative impact on the prognosis of multiple distinct cancers [66–68]. Mutations in the CALR gene have been described in myeloproliferative neoplasms [69–71], causing mislocalization of the corresponding gene product [72], although the exact impact of these mutations on tumor immunosurveillance remains elusive. Regardless, the clinical data validate the importance of the CALR exposure pathway for tumor biology.
12.5 HMGB1
High molecular group B1 protein (HMGB1) is the most abundant nonhistone chromatin-binding protein [73–75]. HMGB1 is usually found in an exclusively nuclear location yet can translocate to the cytoplasm, for instance, after inhibition of histone deacetylases [76]. Moreover, HMGB1 is usually released from cells that undergo necroptosis or secondary necrosis [77, 78]. Mouse cancer cells in which either RIPK3 or MLKL have been knocked out release lower amounts of HMGB1 in response to anthracyclines than their necroptosis-competent controls [18].
Experiments on tumors implanted in mice revealed that HMGB1 is released from cancer cells upon chemotherapy in vivo [79–83]. Cancer cells from which HMGB1 has been depleted by RNA interference are unable to undergo ICD and become resistant to chemotherapy in vivo. Similarly, injection of neutralizing anti-HMGB1 antibodies abolished the anticancer immune response elicited by ICD-inducing chemotherapy in vivo and hence compromised tumor growth reduction [81]. These results support the importance of extracellular HMGB1 as a DAMP in tumor immunology.
Once present in the extracellular space, HMGB1 can interact with multiple additional factors including nucleic acids and bacterial polysaccharides [84–88]. HMGB1 also binds to several receptors including toll-like receptor 4 (TLR4), which is expressed on multiple immune cell types including dendritic cells, in which it stimulates maturation and antigen presentation (Fig. 12.4) [81, 89, 90]. Knockout of TLR4, or that of its adaptor MYD88, from the host immune system abolishes the perception of ICD as well as tumor growth reduction by anthracyclines or oxaliplatin [22, 80, 81, 91]. This defect has been linked to a reduced antigen presentation by dendritic cells and can be partially rescued by treatment with the lysosomal inhibitor chloroquine [81].


Fig. 12.4
HMGB1 facilitates antigen presentation by dendritic cells. High-mobility group box 1 (HMGB1), normally secluded in the nucleus, is released at later stages of immunogenic cell death in response to treatments such as anthracycline-based chemotherapy or ionizing irradiation. Extracellular HMGB1 serves as a ligand for TLR4 on dendritic cells (DCs) and triggers a MYD88-dependent signaling that stimulates DC maturation and antigen presentation to cytotoxic T cells (CTLs). TLR4, toll-like receptor 4
In human breast cancer, reduced HMGB1 expression has been linked to the advancement of the disease and increased tumor size [46]. Reduced HMGB1 expression is a negative prognostic feature in breast cancer and correlates with an intratumoral infiltration by fewer CD8+ cytotoxic T lymphocytes and more immunosuppressive populations of FOXP3+ regulatory T cells and CD68+ tumor-associated macrophages [46]. Moreover, there are at least two cancer types in which a loss-of-function allele of TLR4 compromises patient prognosis, namely, (1) breast cancer and (2) colorectal cancer treated with adjuvant chemotherapy based on anthracyclines and oxaliplatin, respectively [92]. These findings underscore the likely importance of the HMGB1/TLR4 interaction for the fate of cancer patients.
In the case of HMGB1-negative cancers, artificial supply of a synthetic TLR4 ligand, dendrophilin, can compensate for the HMGB1 defect and restore anticancer immune responses elicited by chemotherapy in mouse models. Whether such a strategy might also work in cancer patients bearing HMGB1-negative neoplasia remains to be investigated.
12.6 Type-1 Interferons and Chemokines
In response to chemotherapeutics, tumor cells liberate nucleic acids including DNA and double-stranded RNA that may activate intracellular or extracellular sensors for ectopic molecules of this kind. One example for such nucleic acid sensor is the toll-like receptor-3 (TLR3) [93], although other sensors including the GAS/STING pathway might be involved as well [94]. In response to these stimuli that resemble those induced by a viral infection and hence can be referred to as “viral mimicry,” cancer cells transcriptionally activate one or several type-1 interferon genes, secrete the corresponding gene products, and then stimulate their type-1 interferon receptor (IFNAR) to induce a multipronged type-1 interferon response consisting in the activation of multiple antiviral and immunostimulatory gene products (Fig. 12.5) [95]. One quintessential antiviral gene product is myxovirus resistance 1 (MX1), and one well-known immunostimulatory gene product is the C-X-C motif chemokine ligand 10 (CXCL10), which acts on the C-X-C motif chemokine receptor 3 (CXCR3) to attract T lymphocytes into the tumor bed [95]. Cancer cells that lack TLR3, IFNAR, or CXCL10 are unable to elicit anticancer immune responses upon chemotherapy and hence become refractory to the treatment [95]. Local injection of recombinant type-1 interferons and CXCL10 can overcome this defect [95], underscoring the importance of the type-1 interferon response for therapeutic outcome in mouse tumor models.


Fig. 12.5
Type I interferon-dependent chemokine release triggers T cell priming. In response to chemotherapeutics that mimic a viral infection, with regard to the liberation of nucleic acids from the dying cells, sensors for such molecules like the toll-like receptor-3 (TLR3), transcriptionally activate one or several type-1 interferon genes. Once secreted, the interferon stimulates the type-1 interferon receptor (IFNAR) to induce a multipronged type-1 interferon response including the production of C-X-C motif chemokine ligand 10 (CXCL10), which acts on the C-X-C motif chemokine receptor 3 (CXCR3) to recruit T lymphocytes into the tumor bed finally leading to a γδ T cell-mediated priming of αβ T cells. CXCR3, CXC-chemokine receptor 3; IFNAR1; interferon α/β-receptor subunit 1; IL, interleukin
At least in breast cancer patients, the aforementioned pathway seems to be therapeutically relevant. Thus, MX1 expression is induced by chemotherapy in vivo. The absence of signaling through IFNAR, indicated by the lack of signal transducer and activator of transcription 1 (STAT1) phosphorylation [96] or low MX1 expression, constitutes a poor prognostic feature, in particular in the context of anthracycline-based adjuvant chemotherapy [95]. Moreover, a polymorphism that affects the function of TLR3 reportedly influences the fate of breast cancer patients [97]. These results have to be interpreted in the context of mounting clinical evidences that type-1 interferons can be injected into patients to stimulate anticancer immune responses in the context of renal cancer and chronic myeloid leukemia (CML) [98].
12.7 Concluding Remarks and Perspective
As mentioned above, there are multiple DAMPs (such as ANXA1, ATP, CALR, HMGB1, and type-1 interferons) that function as adjuvant signals in the context of immunogenic chemotherapies (Fig. 12.6). It is important to note that these DAMPs do not act in a redundant fashion (in which case they would be able to replace each other) but in a non-redundant way, meaning that removal of one single DAMP (or its receptor) from the system is sufficient to undermine anticancer immunosurveillance elicited by immunogenic chemotherapies. One possibility to look at this problem is to postulate that each of the DAMPs must come into action following a defined spatiotemporary sequence, perhaps within a narrow range of intensity, following the “key-lock principle” [99]. Only if the DAMPs are expressed in the correct order, at the correct intensity, they are able to form the “key” that opens the vault that normally precludes an immune response [99]. Speculatively, this particular design of the system may reduce the probability of unwarranted autoinflammatory and autoimmune reactions in normal tissues [100]. On the other hand, this means that suppression of one single DAMP due to mutation (perhaps driven by immunoselection) or inhibition of one single DAMP receptor is sufficient to subvert anticancer immunosurveillance and to reduce the chance of cancer patients to control their disease upon chemotherapy.


Fig. 12.6
Mechanisms of immunogenic cell death in therapy-induced immunosurveillance. Cancer cells undergoing immunogenic cell death (ICD) in response to chemotherapeutic treatments, such as doxorubicin or oxaliplatin, exhibit or release certain danger-associated molecular patterns (DAMPs) such as calreticulin (CALR) ATP, type I interferon (IFN), high-mobility group box 1 (HMGB1), and annexin A1 (ANXA1). Ligation of cognate receptors on the surface of myeloid or lymphoid cells facilitates the recruitment and activation of dendritic cells, their homing to the dying cancer cells, subsequent tumor antigen uptake, and final presentation (upon maturation of the dendritic cells). The production of immunostimulatory cytokines eventually leads to the onset of an adaptive immune response involving αβ and γδ T cells that reestablishes cancer immunosurveillance
Irrespective of these speculations, it is possible to measure all known DAMPs in cultured cells exposed to libraries of anticancer agents to identify ICD inducers. In practical terms, this is achieved by generating biosensor cell lines that express fluorescent versions of ANXA1, CALR, or HMGB1 that have been fused to green fluorescent protein (GFP) or its derivatives. ATP release can be measured upon staining with chloroquine. The activation of the type-1 interferon response can be determined by placing GFP under the control of the MX1 promoter. Using this battery of biosensors, it is hence possible to select anticancer agents that stimulate all aspects of ICD. We have successfully used this approach to identify ICD inducers that are effective in stimulating anticancer immune responses in vivo, in mouse models [101–103].
It is tempting to speculate that such an approach may become even more useful in selecting successful anticancer drugs based on their ICD-stimulatory potential. Obviously, this approach would require additional in vivo experimentations in preclinical models while carefully avoiding the use of immunodeficient mice carrying xenotransplants like it was done in the past (see Sect. 12.1). Rather, anticancer drug candidates should always be evaluated in immunocompetent rodent models, including humanized mouse models. It is tempting to predict that this kind of approach will greatly reduce the attrition rate that has been characterizing the traditional drug development pipeline.
Acknowledgments
GK is supported by the French Ligue contre le Cancer (équipe labellisée); Agence National de la Recherche (ANR) – Projets blancs; ANR under the frame of E-Rare-2, the ERA-Net for Research on Rare Diseases; Association pour la recherche sur le cancer (ARC); Cancéropôle Ile-de-France; Institut National du Cancer (INCa); Institut Universitaire de France; Fondation pour la Recherche Médicale (FRM); the European Commission (ArtForce); the European Research Council (ERC); the LeDucq Foundation; the LabEx Immuno-Oncology; the SIRIC Stratified Oncology Cell DNA Repair and Tumor Immune Elimination (SOCRATE); the SIRIC Cancer Research and Personalized Medicine (CARPEM); and the Paris Alliance of Cancer Research Institutes (PACRI).
References
1.
Farkona S, Diamandis EP, Blasutig IM. Cancer immunotherapy: the beginning of the end of cancer? BMC Med. 2016;14:73. doi:10.1186/s12916-016-0623-5.PubMedPubMedCentral
3.
Kelland LR. Of mice and men: values and liabilities of the athymic nude mouse model in anticancer drug development. Eur J Cancer. 2004;40(6):827–36. doi:10.1016/j.ejca.2003.11.028.PubMed
4.
Schmoll HJ, Tabernero J, Maroun J, de Braud F, Price T, Van Cutsem E, Hill M, Hoersch S, Rittweger K, Haller DG. Capecitabine plus Oxaliplatin compared with fluorouracil/Folinic acid as adjuvant therapy for stage III Colon cancer: final results of the NO16968 randomized controlled phase III trial. J Clin Oncol. 2015;33(32):3733–40. doi:10.1200/JCO.2015.60.9107.PubMed
5.
Yothers G, O’Connell MJ, Allegra CJ, Kuebler JP, Colangelo LH, Petrelli NJ, Wolmark N. Oxaliplatin as adjuvant therapy for colon cancer: updated results of NSABP C-07 trial, including survival and subset analyses. J Clin Oncol. 2011;29(28):3768–74. doi:10.1200/JCO.2011.36.4539.PubMedPubMedCentral
6.
Andre T, Boni C, Navarro M, Tabernero J, Hickish T, Topham C, Bonetti A, Clingan P, Bridgewater J, Rivera F, de Gramont A. Improved overall survival with oxaliplatin, fluorouracil, and leucovorin as adjuvant treatment in stage II or III colon cancer in the MOSAIC trial. J Clin Oncol. 2009;27(19):3109–16. doi:10.1200/JCO.2008.20.6771.PubMed
7.
Blanke CD, Rankin C, Demetri GD, Ryan CW, von Mehren M, Benjamin RS, Raymond AK, Bramwell VH, Baker LH, Maki RG, Tanaka M, Hecht JR, Heinrich MC, Fletcher CD, Crowley JJ, Borden EC. Phase III randomized, intergroup trial assessing imatinib mesylate at two dose levels in patients with unresectable or metastatic gastrointestinal stromal tumors expressing the kit receptor tyrosine kinase: S0033. J Clin Oncol. 2008;26(4):626–32. doi:10.1200/JCO.2007.13.4452.PubMed
8.
Blanke CD, Demetri GD, von Mehren M, Heinrich MC, Eisenberg B, Fletcher JA, Corless CL, Fletcher CD, Roberts PJ, Heinz D, Wehre E, Nikolova Z, Joensuu H. Long-term results from a randomized phase II trial of standard- versus higher-dose imatinib mesylate for patients with unresectable or metastatic gastrointestinal stromal tumors expressing KIT. J Clin Oncol. 2008;26(4):620–5. doi:10.1200/JCO.2007.13.4403.PubMed
9.
Druker BJ, Guilhot F, O’Brien SG, Gathmann I, Kantarjian H, Gattermann N, Deininger MW, Silver RT, Goldman JM, Stone RM, Cervantes F, Hochhaus A, Powell BL, Gabrilove JL, Rousselot P, Reiffers J, Cornelissen JJ, Hughes T, Agis H, Fischer T, Verhoef G, Shepherd J, Saglio G, Gratwohl A, Nielsen JL, Radich JP, Simonsson B, Taylor K, Baccarani M, So C, Letvak L, Larson RA, Investigators I. Five-year follow-up of patients receiving imatinib for chronic myeloid leukemia. N Engl J Med. 2006;355(23):2408–17. doi:10.1056/NEJMoa062867.PubMed
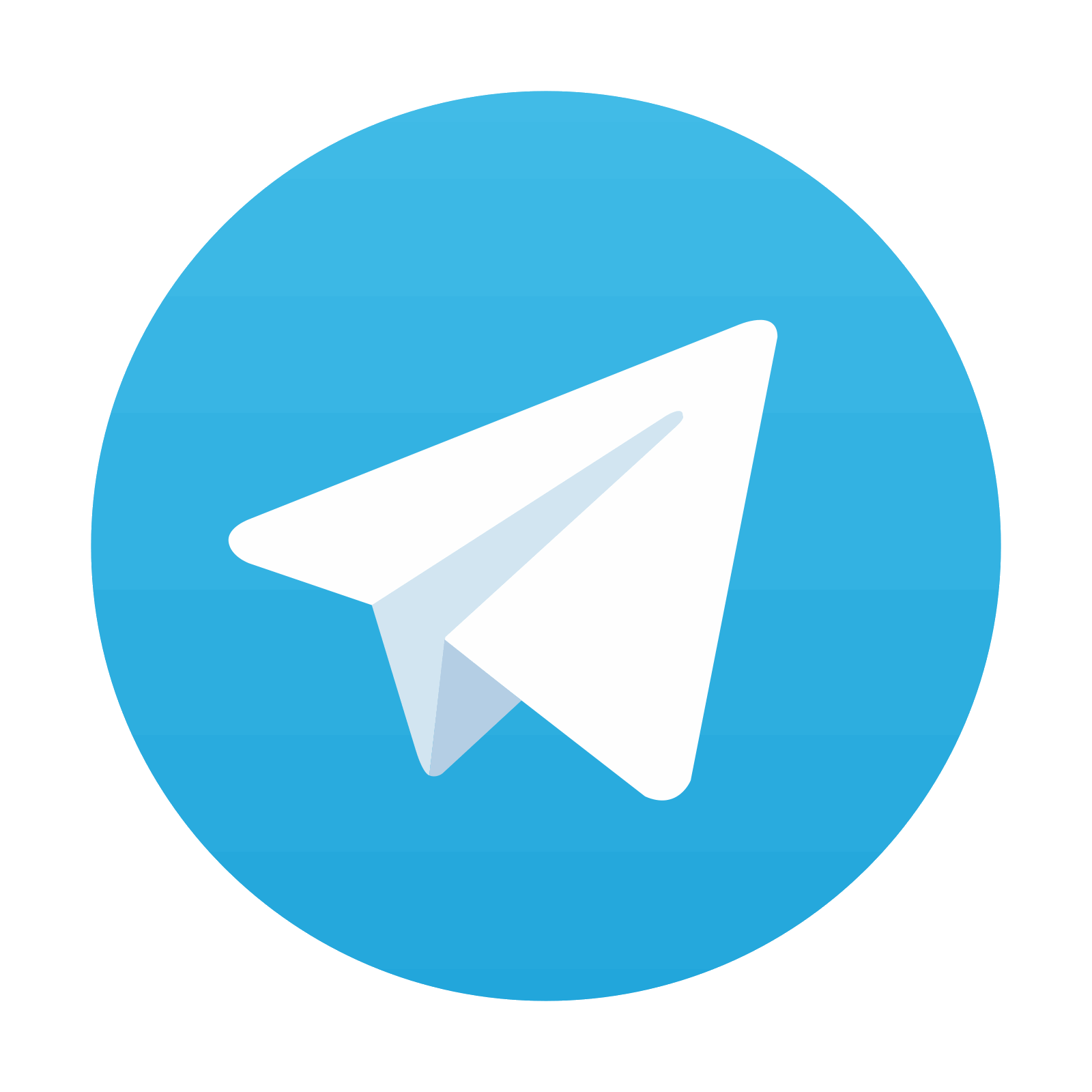
Stay updated, free articles. Join our Telegram channel
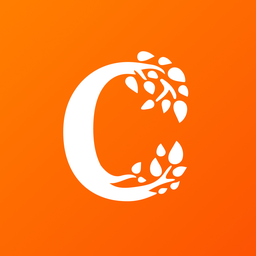
Full access? Get Clinical Tree
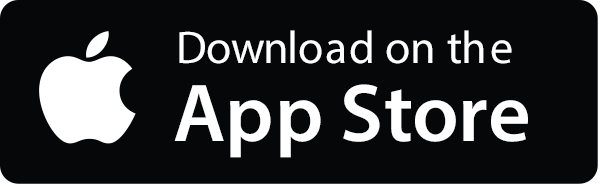
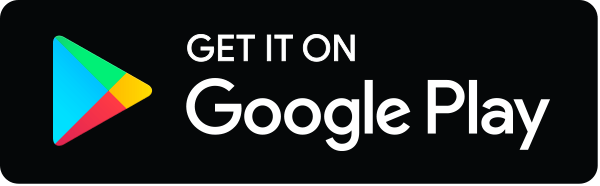