Fig. 3.1
10-year-old boy. 6 months with headaches. 15 days with visual disturbances. Atypical meningioma (WHO II) preoperative imaging and intraoperative extracranial tumor invasion illustration
Patient age, tumor location, and imaging characteristics are key pieces of information for establishing the diagnosis. Supratentorial tumors are more common in neonates and infants up to 2 years old, whereas infratentorial tumors are typically seen in children over 2 years old (Yu et al. 2015) Although some tumors may be found both supra- and infratentorially, tumors that are considered mostly supratentorial and intra-axial include, but are not limited to, astrocytomas, such as diffuse astrocytoma, anaplastic astrocytoma, pleomorphic xanthoastrocytoma (PXA), subependymal giant cell astrocytoma (SEGA), and glioblastoma multiforme (GBM); oligodendrocytoma; primitive neuroectodermal tumor (PNET); dysembryoplastic neuroepithelial tumor (DNET); ganglioglioma; and desmoplastic infantile ganglioglioma. Some supratentorial extra-axial masses include arachnoid cysts, pineal region masses, and choroid plexus tumors.
Conventional Computerized Tomography (CT) and Magnetic Resonance Imaging (MRI) provide tumor characteristics and location information that are paramount in the diagnostic workup. MRI, with and without gadolinium-based contrast, remains the gold standard for neuroimaging in children with suspected CNS lesions. Advanced neuroimaging consists of noninvasive tools for assessing the metabolic and physiologic characteristics of these lesions. Other goals for imaging in the evaluation and treatment of pediatric central nervous system neoplasms include surgical and radiation therapy planning and posttreatment assessment for response.
3.1 Imaging Tools
3.1.1 Computerized Tomography
Advances in CT scanning have greatly improved the ability to detect intracranial neoplasms in a quick and highly replicable manner. Iodinated contrast agents are often utilized to improve characterization of tumors related to their ability to identify alterations in vascular patterns and breakdowns of the blood–brain barrier—both of which are features associated with higher grades of malignancy. However, CT can suffer from beam-hardening artifacts in middle and posterior fossa, and its inability to delineate subtle alteration of tissue affects both its sensitivity and specificity. The physics of CT imaging relies preferentially on changes in electron density for differentiation of normal and abnormal tissue. This is not as robust as the physics involved in the greater tissue differentiation experienced with magnetic resonance imaging and relating to its greater sensitivity and specificity (Hodler et al. 2016).
3.1.2 Magnetic Resonance Imaging
MRI remains the primary tool for characterizing brain tumors, the basic parameters of T1 and T2 relaxation, blood–brain barrier and tissue vascularity assessment with postcontrast imaging, and the diffusion of water molecules in the microarchitecture aid in tumor characterization. Additional MR imaging parameters include various methods of tissue perfusion evaluation, spectroscopic imaging, and blood oxygen level dependent (BOLD) imaging (Hodler et al. 2016).
3.1.2.1 Advanced MRI Applications
Diffusion-Weighted Imaging (DWI), Diffusion-Tensor Imaging (DTI) and Tractography
The addition of a diffusion gradient to an MRI pulse sequence renders acquired images sensitive to the random motion of water along directions parallel to the orientation of the diffusion gradient (Le Bihan and Iima 2015). Early experiments revealed that images in the brain vary significantly with changes in the direction of the applied diffusion gradient: diffusion is highly anisotropic in white matter. In addition to this orientational variability, varying the strength of the gradient (the diffusion weighting, or b-value) sensitizes the image to water diffusing over different distances; stronger gradients encode motion over smaller distances (microns), and weaker gradients are only sensitive to bulk shifts in water over larger distances (millimeters). By systematically varying the strength and direction of the applied diffusion gradients, it is thus possible to collect diffusion data that characterizes the probability of water movement over various spatial scales along each direction in space. Diffusion imaging provides white matter landmarks. Armed with knowledge of the location and trajectory of the principal association, commissural, and projection tracts, the radiologist should use color FA images and fiber tractography to provide clinic-anatomic correlation to the patient’s presenting symptomatology. It is also critical that physicians basing clinical decisions on DTI be familiar with the limitations and potential pitfalls inherent to the technique. DTI can demonstrate different compromise of subcortical fibers. The analysis of the FA values can also grade the compromise of the fibers: displacement, edema, infiltration, or disruption. Comparisons are performed between fibers of both hemispheres and also with normalized tables (Borja et al. 2013; Plaza et al. 2013). Quantitative analyses of the number of fibers, the FA values, and the direction of the fibers can be performed. Additionally, the fMRI provides information about the eloquent cortical brain matter and the DTI, aiding in the determination of the best surgical approach and the extent of subcortical resection to avoid unnecessary fiber injury.
Diffusion-tensor imaging (DTI) has the potential to show the direction of water diffusion (isotropic or anisotropic) to identify white matter tracts and thus to establish a spatial relationship between a white matter tract and the tumor. Quantitative DTI analysis based on a region of interest is conducted in manually selected cross-sectional areas of the main tracts of both hemispheres. For each region of interest, the mean fractional anisotropy (FA), the SD of the mean FA, the mean diffusivity, and the SD of the mean diffusivity are obtained. Major and minor eigenvalues are also estimated. The regions of interest are placed on the internal capsules, superior longitudinal fasciculi, inferior longitudinal fasciculi, inferior occipitofrontal fasciculi, and corpus callosum. Comparisons are performed between hemispheres and with normalized tables.
Diffusion tractography is a development of diffusion-weighted imaging (DWI) that enables identification of the connectivity of specific tracts such as the corticospinal, corpus callosum, arcuate, inferior orbitofrontal, and uncinate tracts (Fig. 3.1) and allows 3D reconstruction of these fibers. A deterministic algorithm based on the connection of voxels surviving arbitrary FA and bending thresholds may be used. Quantitative analyses of the tracts—the number of fibers per tract and the maximum length of each tract—are computed. Lateralization indexes may also be derived from these measures.
The identification of white matter tracts is essential for planning surgery to determine the best surgical approach and the extent of resection to avoid unnecessary fiber injury. DTI and tractography may also help determine the progression or regression of white matter tracts as a result of either tumor growth or resection.
3.1.2.2 MR Perfusion Imaging
Perfusion imaging to determine the hemodynamic parameters of the brain and brain lesions can be performed through either CT or MRI. In children, however, perfusion imaging is limited in most cases to MRI because of the exposure of ionizing radiation from CT examinations. Perfusion MRI evaluates several hemodynamic parameters including cerebral blood volume (CBV), cerebral blood flow (CBF), and mean transit time (MTT); however, CBV has been shown to be the most useful parameter for the evaluation of intracranial masses. More commonly, dynamic susceptibility contrast (DSC) enhanced echo-planar imaging is used for tumor evaluation in the pediatric population. With the use of gadolinium-based contrast agents, differences in local magnetic susceptibility changes (T2*) in the vessels and surrounding tissues are revealed as contrast material passes through these vessels. The amount of signal dropout with the administration of contrast material is detected and the relative CBV (rCBV), CBF, and MTT are calculated and displayed as color maps. Perfusion MRI has improved target selection and increased diagnostic yield with stereotactic biopsy.
Perfusion MRI is a helpful adjunct in differentiating low-grade tumors from high-grade tumors. Typically, high-grade tumors tend to have increased angiogenesis with leaky capillaries and therefore elevated rCBV compared with low-grade tumors. And while processing for rCBV has received the greatest attention among perfusion processing algorithms in differentiating tumor grades in adult tumors (Law et al. 2003), tissue signal-intensity time curves have also demonstrated useful additional diagnostic information including in differentiating primary CNS lymphoma from glioblastoma multiforme and metastases (Mangla et al. 2011). These curves have been shown to have good interobserver agreement for pediatric brain tumors and have also been shown to be useful in identifying low-grade tumor and also sensitive and specific for pilocytic astrocytomas (Ho et al. 2016). Improved target selection and decreased sampling error with stereotactic biopsy have also been described with the use of perfusion MRI to identify and localize the higher-grade component of the tumor. In patients who have undergone treatment, perfusion MRI has been found to be useful for differentiating recurrent tumor from radiation necrosis because recurrent tumor tends to have increased CBV.
Additionally, quantification of vascular permeability (contrast transfer coefficient [Ktrans]) within a tumor can now be obtained through DSC imaging. These Ktrans measurements have been proven useful for grading glioma and may correlate with patient prognosis and survival.
Other relatively newer techniques such as arterial spin labeling (ASL) are also used for perfusion imaging of brain tumors. Although ASL is used primarily in adults, the frequency of this application is increasing in children because ASL is a completely noninvasive technique and requires no contrast administration. In ASL techniques, arterial blood water is magnetically “labeled” using radiofrequency pulses. Two images—one labeled (tag image) and the other non-labeled (control image)—are created and subtracted from each other, resulting in a perfusion contrast image (Paiva et al. 2007).
DSC and ASL may have complementary roles in perfusion imaging of brain tumors. ASL methods use arterial blood water as a freely diffusible tracer perfusion measurement, which makes it more sensitive to absolute quantification of tumor blood flow. DSC, on the other hand, is more sensitive to changes in permeability and capillary blood volume and can be used to image permeability changes in brain.
3.1.2.3 Magnetic Resonance Spectroscopy (MRS)
MRS provides in vivo biochemical and cellular metabolite analyses of tissue. There are five predominant metabolite peaks in proton MRS: choline containing compounds, which reflect membrane turnover; creatine, which represents energy synthesis and serves as an internal control for determining metabolite ratios given its relative stability; N-acetyl aspartate (NAA), which is found mostly in neurons but may also be found in glial cells and serves mostly as a marker of neuronal cells; lactate (Fig. 3.2), which results from anaerobic metabolism and is seen in necrotic tumors and hypoxic or infarcted tissue; and lipid, which peaks when there are increased cellular and myelin breakdown products or nonviable necrotic tissue. Other metabolites such as myoinositol (a glial cell marker) can also be detected. The spectroscopic hallmark of brain tumors relative to normal brain is elevated choline and decreased NAA levels.


Fig. 3.2
Magnetic resonance spectroscopy of a ganglioglioma. Axial enhanced T1 and axial T2 images are demonstrating a right parietal intra-axial hyper intense lesion. MRS is demonstrating elevation of choline and lactate, compatible with a ganglioglioma
MRS is useful in the evaluation of brain tumors in pediatric patients by helping determine the diagnosis, grade, and extent of the tumor. MRS can also differentiate radiation necrosis from tumor recurrence because normal metabolite levels after treatment favor edema and postsurgical changes. Quantitative, rather than qualitative, MRS has been recently suggested for the assessment of the metabolic profiles of pediatric brain tumors. Work has also shown that incorporating functional MRI and spectroscopic imaging into the radiation treatment planning process was feasible and resulted in significant changes in target location and volumes compared with anatomic imaging alone. A major benefit of this strategy was that higher-grade elements within the low-grade gliomas could be more appropriately targeted (Narayana et al. 2007). MRS provides in vivo biochemical and cellular metabolite analyses of brain tissue. It is useful in determining the diagnosis, grade, and extent of the tumor (Panigrahy and Bluml 2009). Additionally, MRS can differentiate radiation necrosis from tumor recurrence.
3.1.2.4 Functional MRI (fMRI)
Mapping the language function before brain tumor resection is crucial for preventing postsurgical deficits and maximizing restoration of language function following surgery (Duffau 2007). Preoperative language mapping via task-fMRI provides noninvasive functional localization of eloquent cortex by contrasting the blood oxygenation level-dependent (BOLD) signal changes between the task and baseline state (Fox et al. 1984; Ogawa et al. 1992). However, its effectiveness is often limited by patient’s cognitive ability and task performance (Pujol et al. 1998). fMRI is able to localize eloquent cortical gray matter that controls language, motor, and memory functions by imaging the local changes in CBF when activated or stimulated. This process is necessary for achieving a maximal safe resection of tumors located in cortical eloquent areas. It can determine the extent of resection according to the location of functional areas. Given the increasing attention to both early and late radiation effects to normal tissue, particularly in children (Marx et al. 1999), techniques such as fMRI to improve planning strategies are gaining increasing attention (Fig. 3.3). There is continued investigation in using fMRI in radiotherapy planning to define functional areas at risk when high doses are considered along tracts in the region of a lesion (Garcia-Alvarez et al. 2006).


Fig. 3.3
fMRI motor tasking. Coronal FLAIR imaging of the head showing BOLD acquisitions of motor tasking, including (a) the toes and (b) hand in relation to an intra-axial mesial frontal lesion
3.1.3 Vascular Imaging
Angiographic evaluations such as CT angiography (CTA) and MR angiography (MRA) remain relevant for pretreatment planning in highly vascularized neoplasms (Vogl et al. 1992). Digital subtraction catheter based angiography also remains relevant despite advances in CTA and MRA, but it is typically reserved for cases with possible preoperative embolization (Wang et al. 2013). CTA is widely available, minimally invasive, and allows for high-resolution rapid assessment of the cerebral arteries. The short scan time also enables evaluation of patients who may be unable to tolerate the longer scan times involved with other modalities, such as critically ill children or those with altered mental status. Furthermore, CTA is less prone to motion-related artifacts in patients compared to flow-related imaging such as MRA (Johnson et al. 2012). Modern multi-detector CT provides rapid scan time, increased resolution, and decreased radiation dose (Mahesh 2002). Unlike MRA, metallic foreign bodies, implanted metallic hardware, such as pacemakers, aneurysm clips, and various orthopedic devices are not a contraindication to CTA. Therefore, a greater percentage of patients are able to undergo imaging with CT compared to MRI.
MRA is a safe, convenient, and noninvasive screening tool for cerebrovascular evaluation. Unenhanced MRA is frequently used to evaluate the cerebral vasculature and is usually performed using time-of-flight (TOF) or phase contrast (PC) flow sensitive imaging techniques, which can be either a two-dimensional (2D) or 3D volumetric acquisition. Although these methods have the benefit of no contrast material administration, there are issues with the diagnostic quality of this technique, particularly in the setting of either pulsation artifacts (e.g., from large vessels), diminutive vessels, or in the case of slow flow. These sequences are also vulnerable to signal-intensity dropout artifacts in stenotic vascular segments that can cause issues with distinguishing near from total occlusion (Mustert et al. 1998; Nederkoorn et al. 2002).
The addition of intravenous gadolinium for contrast-enhanced MRA (CE-MRA) helps to overcome the limitations seen with unenhanced MRA; however, it also adds to the cost and complexity of imaging (Carr et al. 2001; Leclerc et al. 1999; Yang et al. 2005). CE-MRA is increasingly used for evaluation of the carotid and vertebral arteries due to high sensitivity and specificity compared with DSA, but it does not offer enough significant advantages to be routinely used for intracranial vascular evaluation. Surgical blood loss that would be of little concern for an adult can represent a much more significant risk in pediatric patients, as their total blood volume is much lower. Intraoperative MRI (structural sequences, DTI, fMRI) has improved surgical precision for making accurate approaches without injury to eloquent or deep cortical structures (Fan et al. 2016; Jolesz 2005).
3.1.4 Positron Emission Tomography (PET)
Although it provides a direct and multiplanar visualization, MR imaging may present some limitations in surgical planning such as in accurately delineating tumor margins. Pediatric brain tumors may appear to be particularly infiltrative on MR imaging, especially on T1-weighted sequences with or without Gd. Enhancement may be discrete or heterogeneous (as with oligodendrogliomas and fibrillary astrocytomas) and does not always provide precise delineation for complete image-guided resection. MR imaging modalities have been shown to be inaccurate in identifying tumor boundaries in many low- and high-grade gliomas in both children and adults. The sensitivity and specificity for detecting tumor tissue have been reported to be 96 and 53%, respectively; those for detecting anaplastic tissue have been reported to be 72 and 65%, respectively (Plathow and Weber 2008). The accuracy of MR imaging is also limited in detecting residual tumor immediately after surgery (even when T1-weighted, T2-weighted, and FLAIR imaging sequences are obtained within 3 days), in delineating abnormal residual signals, and in differentiating residual tumor signals from inflammatory reactions. As the early confirmation of a complete resection represents a key prognostic factor and allows for early second-look surgery, the value of early postoperative imaging is crucial. A final limitation of MR imaging is the inaccuracy of T1-weighted images with or without Gd-DTPA sequences in identifying anaplastic tissue in histologically heterogeneous brain tumors, especially gliomas. Therefore, MR imaging guidance may either lead stereotactic biopsies to inaccurate targeting and sampling, suboptimal diagnostic yield, and underestimated tumor grading, or lead navigation-based volumetric resections to inaccurately target tumor parts presenting with the highest evolving potential.
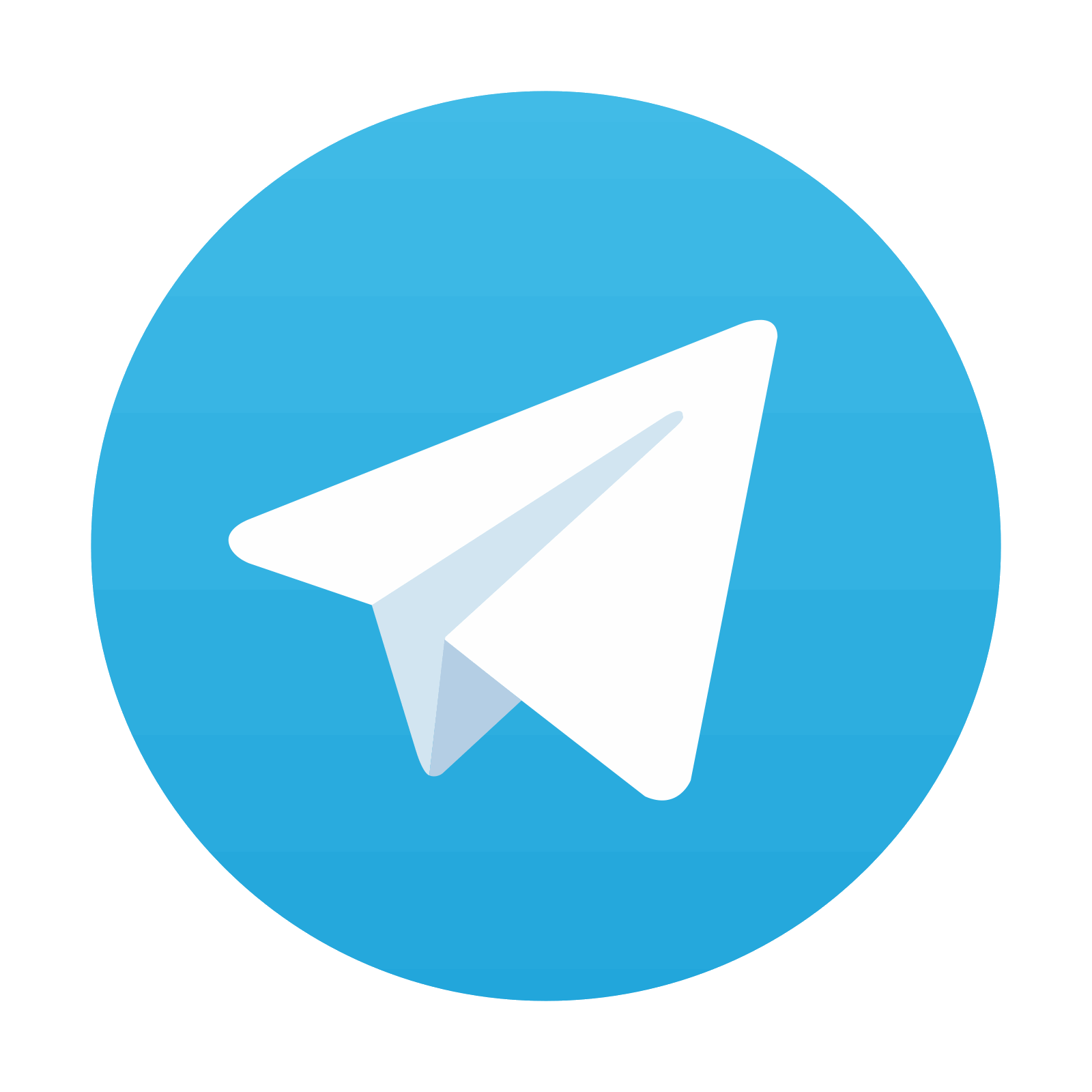
Stay updated, free articles. Join our Telegram channel
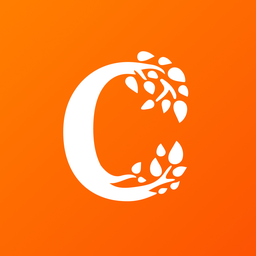
Full access? Get Clinical Tree
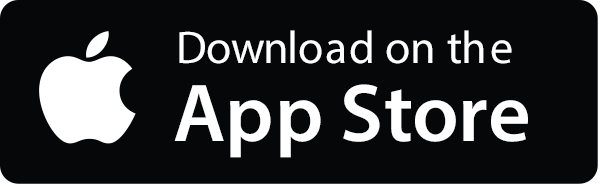
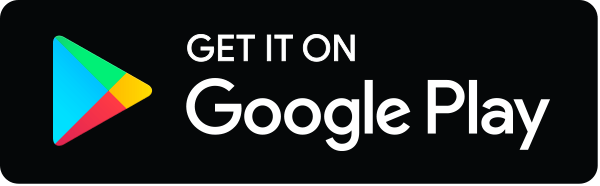