Fig. 24.1
(a) Electronic portal imaging device (EPID)-based imaging (b) compared to the digitally reconstructed radiograph (DRR) from the planning image set for whole brain treatment fields for a 15-year-old patient managed with craniospinal irradiation
In most cases, the target structure itself cannot be localized on planar imaging due to lack of adequate contrast for soft tissue delineation by this modality. Thus, localization for planar-based brain IGRT is generally directed toward bony or metal surrogates (Alcorn et al. 2014a); particularly for proton-based brain radiotherapy, fiducials may be inserted into the outer table of the skull to improve localization (Chen et al. 2007). As treatment is generally targeted as at a surrogate with planar imaging, changes in target conformation or location over time may not be readily detected. Moreover, the two-dimensional nature of planar imaging does not allow for assessment of rotational error (Goyal and Kataria 2014).
24.2.2 CT-Based IGRT
The most common modality used for pediatric brain IGRT is CT imaging (Alcorn et al. 2014a). Images may be generated by devices located on the linear accelerator such as with cone-beam CT (CBCT) or by off-board equipment such as with CT-on-Rails units. With CBCT, an attached X-ray imaging system acquires multiple kV- or MV-planar radiographs as the gantry rotates, and an algorithm then reconstructs these radiographs into volumetric images (Jaffray et al. 2002). With CT-on-Rails, the treatment table is physically moved between a kV-CT scanner for imaging and a gantry for treatment (de Crevoisier et al. 2006; Goyal and Kataria 2014). For Tomotherapy units, the treatment beam itself is used to create MV-CT images for IGRT (Ruchala et al. 1999; Goyal and Kataria 2014). CBCT technology for proton therapy is also being developed (Engelsman et al. 2013).
Although better than planar-based IGRT, there is generally still insufficient soft tissue contrast to visualize the target volume on CT-based IGRT for brain treatment. As such, bony anatomy or metallic clips or markers are generally used as surrogates for alignment purposes. Moreover, CT-based IGRT cannot be performed concurrently with radiation treatment delivery, as the photons from the treating beam would interfere with image acquisition. As such, intrafractional error cannot be corrected in real-time when the treatment beam is on.
24.2.3 MRI-Based IGRT
Although uncommonly used in clinical practice to date, MRI-based IGRT may be particularly useful in the treatment of pediatric brain tumors. First, the improved soft tissue distinction afforded by MRI may allow for better target localization for brain targets. Additionally, MRI does not utilize ionizing radiation, thus reducing the total radiation dose delivered as compared to planar- or CT-based IGRT. Moreover, available systems can perform real-time image guidance during radiation delivery, allowing for intrafractional adjustments. Of note, MRI-based IGRT is particularly susceptible to artifacts from motion and metal densities (Goyal and Kataria 2014) and may require anesthesia for the treatment of pediatrics.
24.2.4 Other IGRT Modalities
Additional means for IGRT include ultrasound and PET imaging as well as optical, infrared, and electromagnetic tracking systems (Goyal and Kataria 2014). Ultrasound is an attractive modality for pediatric IGRT because it lacks ionizing radiation and could potentially be used for real-time target localization. However, inadequate penetration of ultrasound through intact bone limits its use in the treatment of brain tumors after fusion of the fontanelles has occurred. Similarly, although PET systems mounted to the treatment unit have been described (Nishio et al. 2010), they may be of limited utility for brain tumors, where background PET-avidity of the brain parenchyma reduces the ability to delineate the target volume. Use of infrared and optical tracking is not reported for pediatric brain IGRT. Electromagnetic tracking would require surgical insertion of transponders into the brain, reducing its appeal for the treatment of brain targets.
24.2.5 Image Guidance Procedure
The general procedure for patient positioning with 3D-imaging based IGRT is outlined in Fig. 24.2. The patient is positioned on the treatment table, usually by means of an immobilization device with or without alignment to surface markers. The image guidance scan (such as a CBCT) is obtained, and the treatment site is identified either by direct visualization of the target or by means of a surrogate (e.g., nearby bony structure, fiducial marker). The image guidance scan is compared to images derived from the treatment planning scan such as digitally reconstructed radiographs (DRR). Physical shifts in patient positioning on the treatment table are then performed such that the treatment area visualized on the image guidance scan aligns with its location on the corresponding images from the treatment planning. As such, the isocenter for treatment delivery is shifted. Additional image guidance scans may be undertaken to verify patient position before, during, or after treatment. Images obtained comparing kV-CBCT to DRR are shown in Fig. 24.3.



Fig. 24.2
(a) The target volume is identified on image guidance scan (dark sphere) and is compared to images from the treatment planning image set (white sphere). (b) The treatment table and/or patient are physically shifted in the x, y, and z translational directions and rotated such that the positions of these volumes are aligned (c). Figure courtesy of Jacob S.J. Joseph

Fig. 24.3
(a) AP and (b) lateral kilovoltage cone-beam CT (kV-CBCT) performed for a supratentorial tumor boost using IMRT for the same patient as in Fig. 24.1. The PTV is outlined in purple. The kV-CBCT image is overlaying the planning CT in a checkerboard pattern after the alignment procedure in Fig. 24.2 was performed. The kV-CBCT images are marked with red asterisks. Increased scatter in kV-CBCT image set augments noise and decreases image quality as compared to the planning CT. Nonetheless, note that the bony anatomy is well visualized on both the kV-CBCT and planning CT images
Stereotactic brain radiotherapy with both photons and protons requires additional setup steps to ensure adequate target delineation given relatively large fractional doses, rapid dose fall-off, and the use of small or no treatment margins. For units utilizing a gamma source, a rigid head frame is generally affixed to the patient, and treatment is delivered on the same day as the planning image set is obtained. Localization is achieved via two coordinates associated with the rigid head frame (Chen et al. 2007). For linear accelerator-based treatment, a thermoplastic mask is standardly used, with imaging for treatment planning performed in advance of the treatment delivery. IGRT for stereotactic radiotherapy using standard linear accelerators typically involves either planar or CBCT alignment as per Fig. 24.3. For robotic linear accelerators, brain-directed IGRT is generally achieved with multiple planar images directed at the skull anatomy. A 2D–3D image registration algorithm is used to align the planar images to the planning image set, and shifts are made in the plan delivery to correct for setup discrepancies and patient movement. 2D images are repeated periodically during the course of treatment to account for intrafractional changes (Fu and Kuduvalli 2008). In proton-based stereotactic radiotherapy, IGRT is generally comprised of kV-planar images targeting either bony anatomy or fiducials within the skull bone (Chen et al. 2007).
24.3 Potential Advantages/Disadvantages
24.3.1 Advantages of IGRT
By improving localization of the target volume, IGRT maximizes precision and accuracy of radiotherapy delivery. This not only improves coverage of the target volume but potentially minimizes the dose to adjacent normal tissue that may have been inadvertently treated without adequate localization. Such precision is of particular importance for brain-directed IGRT, where excess dose to critical and sensitive structures such as the optic apparatus and brainstem can have especially morbid or fatal effects. For example, Beltran et al. (2012) showed that relatively small rotational errors (≥4°) in the CNS could result in significant changes in dose to the PTV as well as adjacent serial organs.
Moreover, IGRT allows for the use of smaller treatment margins. Per the ICRU definition, the CTV-to-PTV margin accounts for both internal organ motion and patient setup error (Stroom and Heijmen 2002). Improved target delineation through IGRT allows for positional adjustments to be made to account for internal movement of the target over the course of treatment. Further, increased setup accuracy with IGRT reduces setup variations. By addressing both features of the CTV-to-PTV margin definition, IGRT thus allows for reduction in the margin required to account for these uncertainties (Goyal and Kataria 2014).
In turn, by reducing the margins required, the treatment volume can be reduced. In combination with increasingly conformal radiotherapy techniques, this also allow for dose escalation strategies that target the intended treatment volume while sparing nearly normal tissue (Michalski et al. 2001; Jaffray et al. 2007). This may be particularly relevant for radioresistant tumor types, where such dose escalation has been associated with improved local control (Pollack et al. 2002; Chauvet et al. 2003). Additionally, the premium for increased conformality and smaller volumes may be especially high when treating pediatrics given concern for late toxicity related to both cumulative dose and distribution of dose received.
Equally exciting is the potential for the employment of adaptive radiotherapy protocols with the use of IGRT. Data suggests significant change in both the volume of the target and of adjacent normal structures over the course of radiotherapy in select disease entities. Such variation results in the potential for subsequent error in the actual dose delivered versus the dose estimated at the time of initial planning (Bucci et al. 2007; Frank et al. 2008). Adaptive radiotherapy seeks to address this problem through alteration in the treatment plan over the course of radiotherapy. Such alterations could help to account for tumor growth or shrinkage and changes to organ motion or patient weight that develop over the course of treatment. These types of changes are of particular importance since deformation of the target volume or other structures of interest may not be correctable with simple rigid couch shifts performed during routine IGRT (Dong 2012). Additionally, by further addressing internal motion and setup errors, adaptive radiotherapy may allow for further reduction in CTV-to-PTV margins.
Techniques for optimal delivery of adaptive radiotherapy remain investigational; one promising approach relies on using deformable image registration techniques to fuse CBCT images to planning CT image. The cumulative dose to the target and other organs is then recalculated, and altered treatment volumes are applied (Dong 2012). An additional means for adaptive planning utilizes functional imaging such as PET and MRI to direct treatment to specific physiologic changes observed within the target over the course of radiotherapy (Cao 2011). Particularly relevant to the pediatric population is an adaptive radiotherapy protocol for craniopharyngioma reported by Beltran et al. in which weekly MR imaging and replanning were used to account for changes in target volume caused by fluctuations in cyst size during radiotherapy (Beltran et al. 2009). Otherwise, applications of these techniques to the pediatrics population have not been extensively published to date.
An additional benefit of IGRT is that it provides a means for estimation of the margins necessary to account for treatment uncertainties. Comparison of alignment between serial scans approximates inter-fraction error. Comparison of pre- and post-treatment imaging indicates the degree of intrafractional error. Inter- and intrafractional errors combine to estimate total random error. Inter-patient and group mean error estimate systematic error. These two values form the basis of popular recipes used to define adequate treatment margins across a population (Van Herk 2004, 2011).
24.3.2 Disadvantages of IGRT
Despite these benefits, there are a number of important disadvantages to IGRT that must be considered. A primary concern in the use of pediatric brain IGRT is the lack of prospective evidence supporting the efficacy of IGRT in this patient population, particularly when combined with increasingly conformal plans and reduced margins. Because recurrence in brain sites may be especially morbid, the cost of in-field and marginal misses may be especially high. Moreover, implementation of IGRT strategies requires specialized training for radiation therapists, physicist, and physicians, creating the risk for inappropriate application and delivery of this technology.
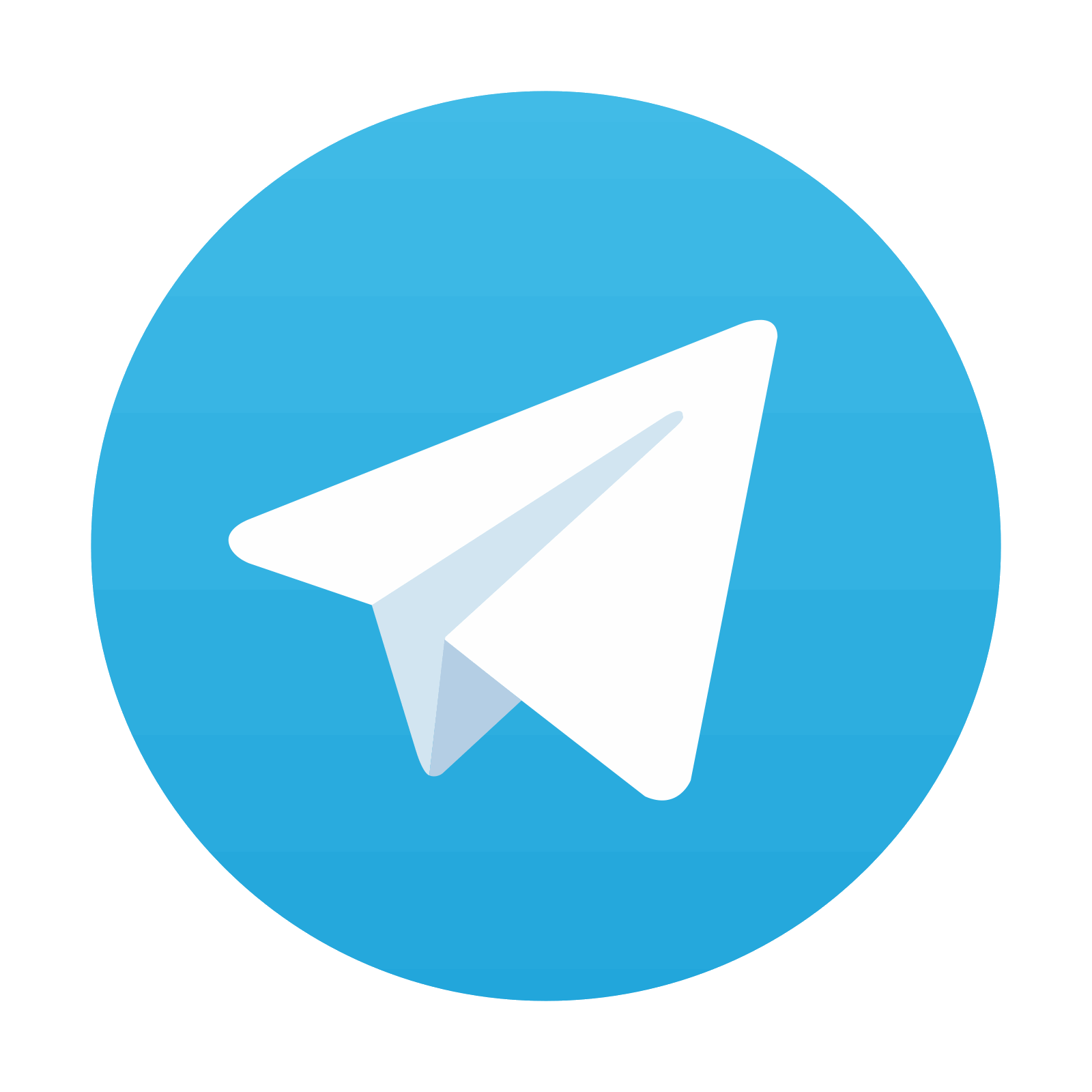
Stay updated, free articles. Join our Telegram channel
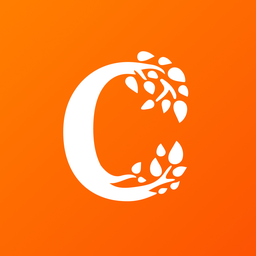
Full access? Get Clinical Tree
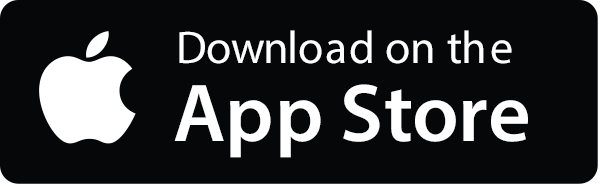
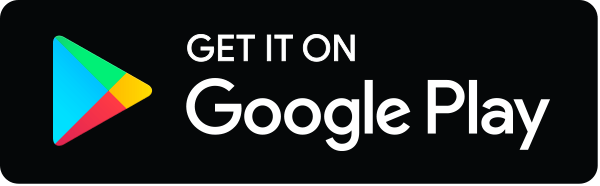