Abbreviations
- ACTH Adrenocorticotropic hormone
- ADH Antidiuretic hormone (vasopressin)
- CLIP Corticotropin-like intermediate lobe peptide
- CRH Corticotropin-releasing hormone
- CRHBP Corticotropin-releasing hormone-binding protein
- FGF8 Fibroblast growth factor 8
- FGFR1 Fibroblast growth factor receptor 1
- FSH Follicle-stimulating hormone
- GABA Gamma-aminobutyric acid
- GH Growth hormone (somatotropin)
- GHBP Growth hormone–binding protein
- GHRH Growth hormone–releasing hormone
- GHS-R Growth hormone secretagogue receptor
- GnRH Gonadotropin-releasing hormone
- hCG Human chorionic gonadotropin
- hMG Human menopausal gonadotropin
- hPL Human placental lactogen
- ICMA Immunochemiluminescent assay
- IGF Insulin-like growth factor
- IRMA Immunoradiometric assay
- KAL1 Kallmann syndrome 1
- LH Luteinizing hormone
- β-LPH β-Lipotropin
- Met-Enk Methionine-enkephalin
- MEN Multiple endocrine neoplasia
- MSH Melanocyte-stimulating hormone
- Pit-1 Pituitary-specific positive transcription factor 1
- POMC Pro-opiomelanocortin
- PROK2 Prokineticin 2
- PROKR2 Prokineticin receptor 2
- Prop-1 Prophet of Pit-1
- PRL Prolactin
- PTTG Pituitary tumor transforming gene
- SHBG Sex hormone–binding globulin
- SIADH Syndrome of inappropriate secretion of antidiuretic hormone
- TRH Thyrotropin-releasing hormone
- TSH Thyroid-stimulating hormone (thyrotropin)
- VIP Vasoactive intestinal peptide
Hypothalamus and Pituitary Gland: Introduction
The hypothalamus and pituitary gland form a unit that exerts control over the function of several endocrine glands—thyroid, adrenals, and gonads—as well as a wide range of physiologic activities. This unit is highly conserved across vertebrate species and constitutes a paradigm of neuroendocrinology—brain–endocrine interactions. The actions and interactions of the endocrine and nervous systems, whereby the nervous system regulates the endocrine system and endocrine activity modulates the activity of the central nervous system, constitute the major regulatory mechanisms for virtually all physiologic activities. These neuroendocrine interactions are also important in pathogenesis. This chapter will review the normal functions of the pituitary gland, the neuroendocrine control mechanisms of the hypothalamus, and the disorders of those mechanisms.
Nerve cells and endocrine cells, which are both involved in cell-to-cell communication, share certain characteristic features—secretion of chemical messengers (neurotransmitters or hormones) and electrical activity. A single chemical messenger—peptide or amine—can be secreted by neurons as a neurotransmitter or neural hormone and by endocrine cells as a classic hormone. Examples of such multifunctional chemical messengers are shown in Table 4–1. The cell-to-cell communication may occur by four mechanisms: (1) autocrine communication via messengers that diffuse in the interstitial fluid and act on the cells that secreted them, (2) neural communication via synaptic junctions, (3) paracrine communication via messengers that diffuse in the interstitial fluid to adjacent target cells (without entering the bloodstream), and (4) endocrine communication via circulating hormones (Figure 4–1). The two major mechanisms of neural regulation of endocrine function are direct innervation and neurosecretion (neural secretion of hormones). The adrenal medulla, kidney, parathyroid gland, and pancreatic islets are endocrine tissues that receive direct autonomic innervation (see Chapters 9, 10, 11). An example of neurosecretory regulation is the hormonal secretion of certain hypothalamic nuclei into the portal hypophysial vessels, which regulate the hormone-secreting cells of the anterior lobe of the pituitary. Another example of neurosecretory regulation is the posterior lobe of the pituitary gland, which is made up of the endings of neurons whose cell bodies reside in hypothalamic nuclei. These neurons secrete vasopressin and oxytocin into the general circulation.
Neurotransmitter (Present in Nerve Endings) | Hormone Secreted by Neurons | Hormone Secreted by Endocrine Cells | |
---|---|---|---|
Dopamine | + | + | + |
Norepinephrine | + | + | + |
Epinephrine | + | + | |
Somatostatin | + | + | + |
Gonadotropin-releasing hormone (GnRH) | + | + | + |
Thyrotropin-releasing hormone (TRH) | + | + | |
Oxytocin | + | + | + |
Vasopressin | + | + | + |
Vasoactive intestinal peptide | + | + | |
Cholecystokinin (CCK) | + | + | |
Glucagon | + | + | |
Enkephalins | + | + | |
Pro-opiomelanocortin derivatives | + | + | |
Other anterior pituitary hormones | + | + |
The anatomic relationships between the pituitary and the main nuclei of the hypothalamus are shown in Figure 4–2. The posterior lobe of the pituitary (neurohypophysis) is of neural origin, arising embryologically as an evagination of the ventral hypothalamus and the third ventricle. The neurohypophysis consists of the axons and nerve endings of neurons whose cell bodies reside in the supraoptic and paraventricular nuclei of the hypothalamus and supporting tissues. This hypothalamic-neurohypophysial nerve tract contains approximately 100,000 nerve fibers. Repeated swellings along the nerve fibers ranging in thickness from 1 to 50 μ m constitute the nerve terminals.
The human fetal anterior pituitary anlage is initially recognizable at 4 to 5 weeks of gestation, and rapid cytologic differentiation leads to a mature hypothalamic-pituitary unit at 20 weeks. The anterior pituitary (adenohypophysis) originates from Rathke pouch, an ectodermal evagination of the oropharynx, and migrates to join the neurohypophysis. The portion of Rathke pouch in contact with the neurohypophysis develops less extensively and forms the intermediate lobe. This lobe remains intact in some species, but in humans its cells become interspersed with those of the anterior lobe and develop the capacity to synthesize and secrete pro-opiomelanocortin (POMC) and adrenocorticotropic hormone (ACTH). Remnants of Rathke pouch may persist at the boundary of the neurohypophysis, resulting in small colloid cysts. In addition, cells may persist in the lower portion of Rathke pouch beneath the sphenoid bone, the pharyngeal pituitary. These cells have the potential to secrete hormones and have been reported to undergo adenomatous change.
The pituitary gland itself lies at the base of the skull in a portion of the sphenoid bone called the sella turcica (Turkish saddle). The anterior portion, the tuberculum sellae, is flanked by posterior projections of the sphenoid wings, the anterior clinoid processes. The dorsum sellae forms the posterior wall, and its upper corners project into the posterior clinoid processes. The gland is surrounded by dura, and the roof is formed by a reflection of the dura attached to the clinoid processes, the diaphragma sellae. The arachnoid membrane and, therefore, cerebrospinal fluid are prevented from entering the sella turcica by the diaphragma sellae. The pituitary stalk and its blood vessels pass through an opening in this diaphragm. The lateral walls of the gland are in direct apposition to the cavernous sinuses and separated from them by dural membranes. The optic chiasm lies 5 to 10 mm above the diaphragma sellae and anterior to the stalk (Figure 4–3).
The size of the pituitary gland, of which the anterior lobe constitutes two-thirds, varies considerably. It measures approximately 15 × 10 × 6 mm and weighs 500 to 900 mg; it may double in size during pregnancy. The sella turcica tends to conform to the shape and size of the gland, and for that reason there is considerable variability in its contour.
The anterior pituitary is the most richly vascularized of all mammalian tissues, receiving 0.8 mL/g/min from a portal circulation connecting the median eminence of the hypothalamus and the anterior pituitary. Arterial blood is supplied from the internal carotid arteries via the superior, middle, and inferior hypophysial arteries. The superior hypophysial arteries form a capillary network in the median eminence of the hypothalamus that recombines in long portal veins draining down the pituitary stalk to the anterior lobe, where they break up into another capillary network and re-form into venous channels. The pituitary stalk and the posterior pituitary are supplied directly from branches of the middle and inferior hypophysial arteries (see Figures 4–2 and 4–3).
Venous drainage of the pituitary, the route through which anterior pituitary hormones reach the systemic circulation, is variable, but venous channels eventually drain via the cavernous sinus posteriorly into the superior and inferior petrosal sinuses to the jugular bulb and vein (Figure 4–4). The axons of the neurohypophysis terminate on capillaries that drain via the posterior lobe veins and the cavernous sinuses to the general circulation. The hypophysial portal system of capillaries allows control of anterior pituitary function by the hypothalamic hypophysiotropic hormones secreted into the portal hypophysial vessels. This provides a short, direct connection to the anterior pituitary from the ventral hypothalamus and the median eminence (Figure 4–5). There may also be retrograde blood flow between the pituitary and hypothalamus, providing a possible means of direct feedback between pituitary hormones and their neuroendocrine control centers.
Figure 4–4
Venous drainage of the pituitary gland—the route by which adenohypophysial hormones reach the systemic circulation.
(Reproduced, with permission, from Findling JW, et al. Selective venous sampling for ACTH in Cushing’s syndrome: differentiation between Cushing’s disease and the ectopic ACTH syndrome. Ann Intern Med. 1981;94:647).
Figure 4–5
Secretion of hypothalamic hormones. The hormones of the posterior lobe (PL) are released into the general circulation from the endings of supraoptic and paraventricular neurons, whereas hypophysiotropic hormones are secreted into the portal hypophysial circulation from the endings of arcuate and other hypothalamic neurons (AL, anterior lobe; ARC, arcuate and other nuclei; MB, mamillary bodies; OC, optic chiasm; PV, paraventricular nucleus; SO, supraoptic nucleus).
Anterior pituitary cells were originally classified as acidophils, basophils, and chromophobe cells based on staining with hematoxylin and eosin. Immunocytochemical and electron microscopic techniques now permit classification of cells by their specific secretory products: somatotrophs (growth hormone [GH]-secreting cells), lactotrophs (prolactin [PRL]-secreting cells), thyrotrophs (cells secreting thyroid-stimulating hormone [thyrotropin; TSH]), corticotrophs (cells-secreting ACTH [corticotropin] and related peptides), and gonadotrophs (luteinizing hormone [LH]– and follicle-stimulating hormone [FSH]–secreting cells). The development of the pituitary gland and the emergence of the distinct cell types from common primordial cells are controlled by a limited set of transcription factors, most notably Prop1 and Pit1 (Figure 4–6). The individual hormone-secreting cells emerge in a specific order and from distinct lineages. Abnormalities of pituitary and lineage–specific transcription factors have been associated with the development of hypopituitarism. Although traditionally the pituitary has been conceptualized as a gland with distinct and highly specialized cells that respond to specific hypothalamic and peripheral hormones, it has become clear that local (ie, paracrine) factors also play a role in normal pituitary physiology.
Figure 4–6
Transcription factors involved in the early development of mouse pituitary, including Tpit.
Tpit is expressed on embryonic day E 11.5, followed by expression of POMC-producing cells by E 12.5 (DAX1, dosage sensitive sex-reversal-adrenal hypoplasia congenital critical region on the X chromosome 1; GATA2, GATA-binding protein 2, zinc-finger transcription factor; α-GSU, alpha subunit of pituitary glycoprotein hormones; Hesx1, homeobox gene expressed in embryonic stem cells 1; Isl1, islet 1 transcription factor; LH/FSH, luteinizing hormone/follicle-stimulating hormone; Lhx3/4, LIM-domain transcription factor 3/4; LIF, leukemia inhibiting factor; NE, neural epithelium; Neuro01, neurogenic basic helix-loop-helix transcription factor 01; OE, oral ectoderm; Pax6, paired box containing transcription factor 6; Pit1, pituitary transcription factor 1; PRL, prolactin; Prop1, prophet of Pit1; Ptx1, pituitary homeobox 1; RP, Rathke pouch; SF1, steroidogenic factor 1; Six3, sine oculis-like homeobox transcription factor 3; Tpit, T-box pituitary transcription factor; VH, ventral hypothalamus).
(From Asteria C. T-Box and isolated ACTH deficiency. Eur J Endocrinol. 2002;146:463).
The GH-secreting cells are acidophilic and usually located in the lateral portions of the anterior lobe. Granule size by electron microscopy is 150 to 600 nm in diameter. These cells account for about 50% of the adenohypophysial cells.
The PRL-secreting cell is a second but distinct acidophil-staining cell randomly distributed in the anterior pituitary. These cells account for 10% to 25% of anterior pituitary cells. Granule size averages approximately 550 nm on electron microscopy. There are two types of lactotrophs: sparsely granulated and densely granulated. These cells proliferate during pregnancy as a result of elevated estrogen levels and account for the twofold increase in gland size.
These TSH-secreting cells, because of their glycoprotein product, are basophilic and also show a positive reaction with periodic acid-Schiff stain. Thyrotrophs are the least common pituitary cell type, making up less than 10% of adenohypophysial cells. The thyrotroph granules are small (50-100 nm), and these cells are usually located in the anteromedial and anterolateral portions of the gland. During states of primary thyroid failure, the cells demonstrate marked hypertrophy, increasing overall gland size.
ACTH and its related peptides (see below) are secreted by basophilic cells that are embryologically of intermediate lobe origin and usually located in the anteromedial portion of the gland. Corticotrophs represent 15% to 20% of adenohypophysial cells. Electron microscopy shows that these secretory granules are about 360 nm in diameter. In states of glucocorticoid excess, corticotrophs undergo degranulation and a microtubular hyalinization known as Crooke hyaline degeneration.
LH and FSH originate from basophil-staining cells, whose secretory granules are about 200 nm in diameter. These cells constitute 10% to 15% of anterior pituitary cells, and they are located throughout the entire anterior lobe. They become hypertrophied and cause the gland to enlarge during states of primary gonadal failure such as menopause, Klinefelter syndrome, and Turner syndrome.
Some cells, usually chromophobes, contain secretory granules but do not exhibit immunocytochemical staining for the major known anterior pituitary hormones. These cells have been called null cells; they may give rise to (apparently) nonfunctioning adenomas. Some may represent undifferentiated primitive secretory cells, and others (eg, glia-like or folliculostellate cells) may produce one or more of the many paracrine factors that have been described in the pituitary. Mammosomatotrophs contain both GH and PRL; these bihormonal cells are most often seen in pituitary tumors. Human chorionic gonadotropin is also secreted by the anterior pituitary gland, but its cell of origin and physiologic significance are uncertain. The six known major anterior pituitary hormones are listed in Table 4–2.
Cellular Source and Histologic Staining | Main Hormone Products | Structure of Hormone | Main Functions |
---|---|---|---|
Somatotroph (acidophil) | GH; also known as STH or somatotropin | 191 amino acids, 22-kDa protein, mainly nonglycosylated | Stimulates the production of IGF-1 (the mediator of the indirect actions of GH); also exerts direct actions on growth and metabolism. |
Lactotroph or mammotroph (acidophil) | PRL | 198 amino acids, 23-kDa protein, mainly nonglycosylated (Note: most of the decidually produced PRL is glycosylated) | Stimulation of milk production (protein and lactose synthesis, water excretion, and sodium retention); inhibits gonadotropin; immunomodulator |
Corticotroph (small cells with basophil granules with strong PAS positivity, indicating the presence of glycoproteins) | Derivatives of POMC, mainly ACTH and β-LPH | POMC: glycosylated polypeptide of 134 amino acid residues ACTH: simple peptide of 39 amino acid residues, 4.5 kDa β-LPH: simple peptide of 91 amino acid residues, 11.2 kDa | ACTH: stimulation of glucocorticoids and sex steroids in the zona fasciculata and zona reticularis of the adrenal cortex, inducinghyperplasia and hypertrophy of the adrenal cortex β-LPH: weak lipolytic and opioid actions |
Thyrotroph (large cells with basophil granules with PASpositivity) | TSH | Glycoprotein hormone consisting of a shared α (89 amino acid) and a TSH-specific β (112 amino acid) subunit Total size: 28 kDa | Stimulation of all aspects of thyroid gland function: hormone synthesis, secretion, hyperplasia, hypertrophy, and vascularization |
Gonadotroph (small cells with basophil granules with periodic acid-Schiff positivity) | LH: named after its effect in females; is identical to the ICSH (interstitial cell stimulating hormone) originally described in males | Glycoprotein hormone consisting of a shared α and an LH-specific β (115 amino acid) subunit Total size: 29 kDa | Females: stimulates steroid hormone synthesis in theca interna cells, lutein cells, and hilar cells; promotes luteinization and maintains corpus luteum Males: stimulates steroid hormone production in Leydig cells |
FSH | Glycoprotein hormone consisting of a shared α and an FSH-specific β (115 amino acid) subunit. Total size: 29 kDa | Females: targets the granulosa cells to promote follicular development; stimulates aromatase expression and inhibin secretion Males: targets the Sertoli cells to promote spermatogenesis and to stimulate inhibin secretion |
Hypothalamic Hormones
The hypothalamic hormones can be divided into those secreted into hypophysial portal blood vessels and those secreted by the neurohypophysis directly into the general circulation. The hypothalamic nuclei, their neurohormones, and their main functions are shown in Table 4–3. The structures of the eight major hypothalamic hormones are shown in Table 4–4.
Nucleus | Location | Major Neurohormones or Function |
---|---|---|
Supraoptic | Anterolateral, above the optic tract | ADH: osmoregulation, regulation of ECF volume Oxytocin: regulation of uterine contractions and milk ejection |
Paraventricular | Dorsal anterior periventricular | Magnocellular paraventricular nucleus (PVN): ADH, oxytocin: same functions as above Parvocellular PVN TRH: regulation of thyroid function CRH: regulation of adrenocortical function, regulation of the sympathetic nervous system and adrenal medulla, regulation of appetite ADH: coexpressed with CRH, regulation of adrenocortical function VIP: prolactin-releasing factor (?) |
Suprachiasmatic | Above the optic chiasm, anteroventral periventricular zone | Regulator of circadian rhythms and pineal function (zeitgeber [pacemaker]): VIP, ADH neurons project mainly to the PVN |
Arcuate | Medial basal hypothalamus close to the third ventricle | GHRH: stimulation of growth hormone GnRH: regulation of pituitary gonadotropins (FSH and LH) Dopamine: functions as PIH Somatostatin: inhibition of GHRH release Regulation of appetite (neuropeptide Y, agouti-related transcript, α-MSH, cocaine- and amphetamine-related transcript) |
Periventricular | Anteroventral | Somatostatin: inhibition of growth hormone secretion by direct pituitary action: most abundant SRIF location |
Ventromedial | Ventromedial | GHRH (as above) Somatostatin: inhibition of GHRH release Functions as a satiety center |
Dorsomedial | Dorsomedial | Focal point of information processing: receives input from ventromedial nucleus (VMN) and lateral hypothalamus and projects to the PVN |
Lateral hypothalamus | Lateral hypothalamus | Functions as a hunger center (melanin-concentrating hormone, anorexins) |
Preoptic area | Preoptic area | Main regulator of ovulation in rodents; only a few GnRH neurons in primates |
Anterior hypothalamus | Anterior hypothalamus | Thermoregulation: cooling center Anteroventral third ventricular region: regulation of thirst |
Posterior hypothalamus | Posterior hypothalamus | Thermoregulation: heating center |
Hormone | Structure |
---|---|
Posterior pituitary hormones
| ![]() |
Oxytocin | ![]() |
Hypophysiotropic hormones
| (pyro)Glu-His-Pro-NH2 (pyro)Glu-His-Trp-Ser-Tyr-Gly-Leu-Arg-Pro-Gly-NH2 |
Growth hormone–releasing hormone (GHRH) | Tyr-Ala-Asp-Ala-Ile-Phe-Thr-Asn-Ser-Tyr-Arg-Lys-Val-Leu-Gly-Gln-Leu-Ser- Ala-Arg-Lys-Leu-Leu-Gln-Asp-Ile-Met-Ser-Arg-Gln-Gln-Gly-Glu-Ser-Asn-Gln- Glu-Arg-Gly-Ala-Arg-Ala-Arg-Leu-NH2 |
Prolactin-inhibiting hormone (PIH, dopamine) | ![]() |
Corticotropin-releasing hormone (CRH) | Ser-Gln-Glu-Pro-Pro-Ile-Ser-Leu-Asp-Leu-Thr-Phe-His-Leu-Leu-Arg-Glu-Val- Leu-Glu-Met-Thr-Lys-Ala-Asp-Gln-Leu-Ala-Gln-Gln-Ala-His-Ser-Asn-Arg-Lys- Leu-Leu-Asp-Ile-Ala-NH2 |
The hypophysiotropic hormones that regulate the secretion of anterior pituitary hormones include growth hormone–releasing hormone (GHRH), somatostatin, dopamine, thyrotropin-releasing hormone (TRH), corticotropin-releasing hormone (CRH), and gonadotropin-releasing hormone (GnRH). The location of the cell bodies of the hypophysiotropic hormone–secreting neurons is depicted in Figure 4–7. Most of the anterior pituitary hormones are controlled by stimulatory hormones, but GH and especially PRL are also regulated by inhibitory hormones. Some hypophysiotropic hormones are multifunctional. The hormones of the hypothalamus are secreted episodically and not continuously, and in some cases there is an underlying circadian rhythm.
Figure 4–7
Location of cell bodies of hypophysiotropic hormone-secreting neurons projected on a ventral view of the hypothalamus and pituitary of the rat. (AL, anterior lobe; ARC, arcuate nucleus; BA, basilar artery; IC, internal carotid; IL, intermediate lobe; MC, middle cerebral; ME, median eminence; PC, posterior cerebral; Peri, periventricular nucleus; PL, posterior lobe; PVL and PVM, lateral and medial portions of the paraventricular nucleus; SO, supraoptic nucleus.) The names of the hormones are enclosed in the boxes (SS, somatostatin; DA, dopamine).
(Courtesy of LW Swanson and ET Cunningham Jr.)
GHRH stimulates GH secretion by, and is trophic for, somatotrophs. GHRH-secreting neurons are located in the arcuate nuclei (see Figure 4–2), and axons terminate in the external layer of the median eminence. The major isoform of GHRH is 44 amino acids in length. It was isolated from a pancreatic tumor in a patient with clinical manifestations of GH excess (acromegaly) associated with somatotroph hyperplasia (see discussion later in the chapter). GHRH is synthesized from a larger precursor of 108 amino acids. Other secretory products derived from this precursor have also been found. Full biologic activity of these releasing factors appears to reside in the 1 to 29 amino acid sequence of the amino terminal portion of the molecule. Human GHRH is a member of a homologous family of peptides that includes secretin, glucagon, vasoactive intestinal peptide (VIP), and others. The half-life of GHRH is approximately 3 to 7 minutes.
Somatostatin inhibits the secretion of GH and TSH. Somatostatin-secreting cells are located in the periventricular region immediately above the optic chiasm (see Figure 4–2) with nerve endings found diffusely in the external layer of the median eminence.
Somatostatin, a tetradecapeptide, has been found not only in the hypothalamus but also in the D cells of the pancreatic islets, the gastrointestinal mucosa, and the C cells (parafollicular cells) of the thyroid. The somatostatin precursor has 116 amino acids. Processing of the carboxyl terminal region of preprosomatostatin results in the generation of the tetradecapeptide somatostatin 14 and an amino terminal extended form containing 28 amino acid residues (somatostatin 28). Somatostatin 14 is the major species in the hypothalamus, whereas somatostatin 28 is found in the gut. In addition to its profound inhibitory effect on GH secretion, somatostatin also has important inhibitory influences on many other hormones, including insulin, glucagon, gastrin, secretin, and VIP. This inhibitory hypothalamic peptide plays a role in the physiologic secretion of TSH by augmenting the direct inhibitory effect of thyroid hormone on the thyrotrophs; administration of anti-somatostatin antibodies results in a rise in circulating TSH level. Somatostatin has a half-life of 2 to 3 minutes.
Dopamine, the primary PRL-inhibitory hormone, is found in the portal circulation and binds to dopamine receptors on lactotrophs. It has a short half-life, on the order of 1 to 2 minutes. The hypothalamic control of PRL secretion, unlike that of the other pituitary hormones, is predominantly inhibitory. Thus, disruption of the hypothalamic-pituitary connection by stalk section, hypothalamic lesions, or pituitary autotransplantation increases PRL secretion. Dopamine-secreting neurons (tuberoinfundibular dopaminergic system) are located in the arcuate nuclei, and their axons terminate in the external layer of the median eminence, primarily in the same area as the GnRH endings (laterally) and to a lesser extent medially (see Figure 4–2). The neurotransmitter gamma-aminobutyric acid (GABA) and cholinergic pathways also appear to inhibit PRL release.
The best-studied factor with PRL-releasing activity is TRH (see discussion later), but there is little evidence for a physiologic role. PRL increase associated with sleep, during stress, and after nipple stimulation or suckling is not accompanied by an increase in TRH or TSH. Another hypothalamic peptide, VIP, stimulates PRL release in humans. Serotonergic pathways may also stimulate PRL secretion, as demonstrated by the increased PRL secretion after the administration of serotonin precursors and by the reduction of secretion following treatment with serotonin antagonists.
TRH, a tripeptide, is the major hypothalamic factor regulating TSH secretion. Human TRH is synthesized from a large precursor of 242 amino acids that contains six copies of TRH. TRH-secreting neurons are located in the medial portions of the paraventricular nuclei (see Figure 4–2), and their axons terminate in the medial portion of the external layer of the median eminence. The half-life of TRH is approximately 6 minutes.
CRH, a 41-amino-acid peptide, stimulates the secretion of ACTH and other products of its precursor molecule, POMC. CRH is synthesized from a precursor of 196 amino acids. The half-life of CRH follows a biphasic pattern in plasma lasting approximately 6 to 10 minutes and 40 to 50 minutes. Both antidiuretic hormone (ADH) and angiotensin II potentiate CRH-mediated secretion of ACTH. In contrast, oxytocin inhibits CRH-mediated ACTH secretion. CRH-secreting neurons are found in the anterior portion of the paraventricular nuclei just lateral to the TRH-secreting neurons; their nerve endings are found in all parts of the external layer of the median eminence. CRH is also secreted from human placenta. The level of this hormone increases significantly during late pregnancy and delivery. In addition, a specific CRH-binding protein (CRHBP) has been described in both serum and in intracellular locations within a variety of cells. It is likely that CRHBPs modulate the actions and plasma half-life of CRH. Since the 1990s, three proteins homologous to CRH, termed urocortins, and two different receptors, have been identified. In addition to the role of CRH in the physiologic response to stress, this family of peptides appears to play a significant role in energy balance.
The secretion of LH and FSH is controlled by a single stimulatory hypothalamic hormone, GnRH. This is achieved through differences in the size and frequency of GnRH release as well as feedback from estrogens and androgens; low-frequency pulses favor FSH release while high-frequency pulses result in LH release. GnRH is a linear decapeptide that stimulates only LH and FSH; it has no effect on other pituitary hormones except in some patients with acromegaly and Cushing disease (see discussion later). The precursor of GnRH—proGnRH—contains 92 amino acids. ProGnRH also contains the sequence of a 56-amino-acid polypeptide called GnRH-associated peptide. This secretory product exhibits PRL-inhibiting activity, but its physiologic role is unknown. GnRH-secreting neurons are located primarily in the preoptic area of the anterior hypothalamus, and their nerve terminals are found in the lateral portions of the external layer of the median eminence adjacent to the pituitary stalk (see Figure 4–2). GnRH has a half-life of 2 to 4 minutes.
The hypothalamus is involved in many nonendocrine functions such as regulation of body temperature, thirst, and food intake and is connected with many other parts of the nervous system. The brain itself is influenced by both direct and indirect hormonal effects. Steroid and thyroid hormones cross the blood–brain barrier and produce specific receptor-mediated actions (see Chapters 1, 7, and 9). Peptides in the general circulation, which do not cross the blood–brain barrier, elicit their effects indirectly (eg, insulin-mediated changes in blood–glucose concentration). In addition, communication between the general circulation and the brain may take place via the circumventricular organs, which are located outside the blood–brain barrier (see later). Moreover, hypothalamic hormones in extrahypothalamic brain function as neurotransmitters or neurohormones. They are also found in other tissues where they function as hormones (endocrine, paracrine, or autocrine). For example, somatostatin-containing neurons are widely distributed in the nervous system. They are also found in the pancreatic islets (D cells), the gastrointestinal mucosa, and the C cells of the thyroid gland (parafollicular cells). Somatostatin is not only secreted into the general circulation as well as locally—it is also secreted into the lumen of the gut, where it may affect gut secretion. A hormone with this activity has been called a lumone. Hormones common to the brain, pituitary, and gastrointestinal tract include not only TRH and somatostatin but also VIP and peptides derived from POMC.
Hypothalamic function is regulated both by hormone-mediated signals (eg, negative feedback) and by neural inputs from a wide variety of sources. These nerve signals are mediated by neurotransmitters including acetylcholine, dopamine, norepinephrine, epinephrine, serotonin, GABA, and opioids. The hypothalamus can be considered a final common pathway by which signals from multiple systems reach the anterior pituitary. For example, cytokines that play a role in the response to infection, such as the interleukins, are also involved in regulation of the hypothalamic-pituitary-adrenal axis. This system of immunoneuroendocrine interactions is important in the organism’s response to a variety of stresses.
The hypothalamus also sends signals to other parts of the nervous system. For example, while the major nerve tracts of the magnocellular neurons containing vasopressin and oxytocin terminate in the posterior pituitary, nerve fibers from the paraventricular and supraoptic nuclei project toward many other parts of the nervous system. In the brain stem, vasopressinergic neurons are involved in the autonomic regulation of blood pressure. Similar neurons project to the gray matter and are implicated in higher cortical functions. Fibers terminating in the median eminence permit release of ADH into the hypophysial-portal system; delivery of ADH in high concentrations to the anterior pituitary may facilitate its involvement in the regulation of ACTH secretion. Magnocellular neurons also project to the choroid plexus, where they may release ADH into the cerebrospinal fluid. In addition to magnocellular neurons, the paraventricular nuclei contain cells with smaller cell bodies—parvicellular neurons. Such neurons are also found in other regions of the nervous system and may contain other peptides such as CRH and TRH.
With the growing appreciation of adipose tissue as an endocrine organ, as well as the increasing problem of obesity and its associated health risks, understanding how energy balance and appetite are regulated has become a major topic of study. In 1901, Frohlich observed that some tumors affecting the pituitary and hypothalamus were associated with excess subcutaneous fat and hypogonadism. Subsequent lesioning experiments by Hetherington and Ranson in the 1940s established the hypothalamus as a fundamental site in the regulation of appetite. These experiments introduced the classic dual center model of food intake where the ventromedial hypothalamic nucleus functions as a satiety center and the lateral hypothalamic area serves as a feeding center. Subsequent studies have led to refinements of this model.
Growing evidence points to the pivotal role of the arcuate nucleus in the integration of feeding signals and energy reserves. It has special access to circulating hormones via the underlying median eminence, an area rich with fenestrated capillaries, that is, it is not protected by the blood–brain barrier (see Figure 4–2). Two populations of neurons involved in the regulation of feeding are located within the arcuate nucleus: one that inhibits food intake via the expression of neuropeptides POMC and cocaine- and amphetamine-regulated transcript, and one that stimulates food intake via expression of neuropeptide Y and agouti-related peptide. The arcuate nucleus projects to second-order neuronal populations including the paraventricular nucleus, dorsomedial nucleus, ventromedial nucleus, and lateral hypothalamic area, which then activate downstream pathways controlling appetite and energy expenditure.
Circulating markers of adiposity (leptin, adiponectin, insulin), and hormones from the gastrointestinal tract (ghrelin, peptide YY, glucagon-like peptide 1, cholecystokinin, oxyntomodulin, pancreatic polypeptide) converge on the hypothalamus and brain stem to signal adequacy of short- and long-term energy stores. Alterations in the levels or tissue sensitivity of these hormones may underlie disorders of weight regulation such as obesity, and could prove useful as therapeutic targets (see also Chapter 20).
The circumventricular organs are secretory midline brain structures that arise from the ependymal cell lining of the ventricular system (Figure 4–8). These organs are located adjacent to the third ventricle—subfornical organ, subcommissural organ, organum vasculosum of the lamina terminalis, pineal, and part of the median eminence—and at the roof of the fourth ventricle—area postrema (see Figure 4–8). The tissues of these organs have relatively large interstitial spaces and have fenestrated capillaries which, being highly permeable, permit diffusion of large molecules from the general circulation; elsewhere in the brain tight capillary endothelial junctions prevent such diffusion—the blood–brain barrier. For example, angiotensin II (see Chapter 10) is involved in the regulation of water intake, blood pressure, and secretion of vasopressin. In addition to its peripheral effects, circulating angiotensin II acts on the subfornical organ resulting in an increase in water intake.
Figure 4–8
Circumventricular organs. The neurohypophysis (NH) and adjacent median eminence, the organum vasculosum of the lamina terminalis (OVLT), the subfornical organ (SFO), and the area postrema (AP) are shown projected on a sagittal section of the human brain. The pineal (PI) and the subcommissural organ (SCO) are also shown but probably do not function as circumventricular organs.
(Reproduced, with permission, from Ganong WF. Review of Medical Physiology. 15th ed. McGraw-Hill; 1993).
The pineal gland, considered by the 17th century French philosopher René Descartes to be the seat of the soul, is located at the roof of the posterior portion of the third ventricle. The pineal gland in humans and other mammals has no direct neural connections with the brain except for sympathetic innervation via the superior cervical ganglion. The pineal gland secretes melatonin, an indole synthesized from serotonin by 5-methoxylation and N-acetylation (Figure 4–9). The pineal releases melatonin into the general circulation and into the cerebrospinal fluid. Melatonin secretion is regulated by the sympathetic nervous system and is increased in response to hypoglycemia and darkness. The pineal also contains other bioactive peptides and amines including TRH, somatostatin, GnRH, and norepinephrine. The physiologic roles of the pineal remain to be elucidated, but they appear to involve regulation of gonadal function and development and chronobiologic rhythms.
The pineal gland may be the site of origin of pineal cell tumors (pinealomas) or germ cell tumors (germinomas). Neurologic signs and symptoms are the predominant clinical manifestations; examples include increased intracranial pressure, visual abnormalities, ataxia, and Parinaud syndrome—upward gaze palsy, absent pupillary light reflex, paralysis of convergence, and wide-based gait. Endocrine manifestations result primarily from deficiency of hypothalamic hormones (diabetes insipidus, hypopituitarism, or disorders of gonadal development). Treatment involves surgical removal or decompression, radiation therapy, and hormone replacement (discussed later).
Anterior Pituitary Hormones
The six major anterior pituitary hormones—ACTH, GH, PRL, TSH, LH, and FSH—may be classified into three groups: ACTH-related peptides (ACTH itself, lipotropin [LPH], melanocyte-stimulating hormone [MSH], and endorphins); the somatomammotropin proteins (GH and PRL); and the glycoproteins (LH, FSH, and TSH). The chemical features of these hormones are set forth in Table 4–2.
ACTH is a 39-amino-acid peptide hormone (MW 4500) processed from a large precursor molecule, POMC (MW 28,500). Within the corticotroph, a single mRNA directs the synthesis and processing of POMC into smaller, biologically active fragments (Figure 4–10), which include β-LPH, α-MSH, β-MSH, β-endorphin, and the amino terminal fragment of POMC. Most of these peptides are glycosylated, which accounts for differences in the reporting of molecular weights. These carbohydrate moieties are responsible for the basophilic staining of corticotrophs.
Two of these fragments are contained within the structure of ACTH: α-MSH is identical to ACTH 1 to 13, and corticotropin-like intermediate lobe peptide (CLIP) represents ACTH 18 to 39 (see Figure 4–10). Although these fragments are found in species with developed intermediate lobes (eg, the rat), they are not secreted as separate hormones in humans. β-LPH, a fragment with 91 amino acids (1-91), is secreted by the corticotroph in equimolar quantities with ACTH. Within the β-LPH molecule exists the amino acid sequence for β-MSH (41-58), γ-LPH (1-58), and β-endorphin (61-91).
ACTH stimulates the secretion of glucocorticoids, mineralocorticoids, and androgens—all steroids from the adrenal cortex (see Chapters 9 and 10). The amino terminal end (residues 1-18) is responsible for this biologic activity. ACTH binds to receptors on the adrenal cortex and induces steroidogenesis through a cAMP-dependent mechanism.
The hyperpigmentation observed in states of ACTH hypersecretion (eg, Addison disease, Nelson syndrome) appears to be primarily due to ACTH binding to the MSH receptor, because α-MSH and β-MSH do not exist as separate hormones in humans.
The physiologic function of β-LPH and its family of peptide hormones, including β-endorphin, is not completely understood. However, both β-LPH and β-endorphin have the same secretory dynamics as ACTH.
The development of immunoradiometric and immunochemiluminescent assays (IRMAs and ICMAs, respectively) has provided a sensitive and practical clinical ACTH assay for the evaluation of pituitary-adrenal disorders. The basal morning concentration ranges from 9 to 52 pg/mL (2-11 pmol/L). Its short plasma half-life (7-12 minutes) and episodic secretion cause wide and rapid fluctuations both in its plasma concentration and in that of cortisol.
Although β-LPH has a longer half-life than ACTH and is more stable in plasma, its measurement has not been extensively utilized. Current data suggest that the normal concentration of β-LPH is 10 to 40 pg/mL (1-4 pmol/L).
The physiologic secretion of ACTH is mediated through neural influences by means of a complex of hormones, the most important of which is CRH (Figure 4–11).
Figure 4–11
The hypothalamic-pituitary-adrenal axis, illustrating negative feedback by cortisol (F) at the hypothalamic and pituitary levels. A short negative feedback loop of ACTH on the secretion of corticotropin-releasing hormone (CRH) also exists.
(Reproduced, with permission, from Gwinup G, Johnson B. Clinical testing of the hypothalamic-pituitary-adrenocortical system in states of hypo- and hypercortisolism. Metabolism. 1975;24:777).
CRH stimulates ACTH in a pulsatile manner: diurnal rhythmicity causes a peak before awakening and a decline as the day progresses. The diurnal rhythm is a reflection of neural control and provokes concordant diurnal secretion of cortisol from the adrenal cortex (Figure 4–12). This episodic release of ACTH is independent of circulating cortisol levels (ie, the magnitude of an ACTH impulse is not related to preceding plasma cortisol levels). An example is the persistence of diurnal rhythm in patients with primary adrenal failure (Addison disease). ACTH secretion also increases in response to feeding in both humans and animals.
Many stresses stimulate ACTH, often superseding the normal diurnal rhythmicity. Physical, emotional, and chemical stresses such as pain, trauma, hypoxia, acute hypoglycemia, cold exposure, surgery, depression, and interleukin-1 and vasopressin administration have all been shown to stimulate ACTH and cortisol secretion. The increase in ACTH levels during stress is mediated by vasopressin as well as CRH. Although physiologic cortisol levels do not blunt the ACTH response to stress, exogenous corticosteroids in high doses suppress it.
Negative feedback of cortisol and synthetic glucocorticoids on ACTH secretion occurs at both the hypothalamic and pituitary levels via two mechanisms: fast feedback is sensitive to the rate of change in cortisol levels, whereas slow feedback is sensitive to the absolute cortisol level. The first mechanism is probably nonnuclear; that is, this phenomenon occurs too rapidly to be explained by the influence of corticosteroids on nuclear transcription of the specific mRNA responsible for ACTH. Recent studies suggest fast feedback is mediated by a novel membrane-associated glucocorticoid receptor that stimulates a rapid synthesis and retrograde release of endocannabinoids, thereby suppressing synaptic excitation. Slow feedback, occurring later, may be explained by a nuclear-mediated mechanism and a subsequent decrease in synthesis of ACTH. This latter form of negative feedback is the type probed by the clinical dexamethasone suppression test. In addition to the negative feedback of corticoids, ACTH also inhibits its own secretion (short-loop feedback).
Growth Hormone
GH or somatotropin is a 191-amino-acid polypeptide hormone (MW 21,500) synthesized and secreted by the somatotrophs of the anterior pituitary. Its larger precursor peptide, pre-GH (MW 28,000), is also secreted but has no physiologic significance.
The primary function of GH is promotion of linear growth. Its basic metabolic effects serve to achieve this result, but most of the growth-promoting effects are mediated by insulin-like growth factor 1 (IGF-I; previously known as somatomedin C). The metabolic and biologic effects of GH and IGF-I are shown in Tables 4–5 and 4–6 (see also Chapter 6).
Function, Parameter Group | Function, Parameter Subgroup | GH | IGF-1 |
---|---|---|---|
Carbohydrate metabolism | Glucose uptake in extrahepatic tissues | Decreasea | Increase |
Hepatic glucose output | Increase | Decrease | |
Hepatic glycogen stores | Increase (jointly with glucocorticoids and insulin) | ||
Plasma glucose | Increase | Decrease | |
Insulin sensitivity | Decrease | Increase | |
Lipid metabolism | Lipolysis in adipocytes, plasma-free fatty acid levels | Increase | Decrease |
Plasma ketone bodies | Increase | Decrease | |
Protein metabolism (muscle, connective tissue) | Amino acid uptake Protein synthesis Nitrogen excretion | Increase (?) Increase (?) Decrease (?) | Increase Increase Decrease |
Target, Source | Parameter | Effect |
---|---|---|
Blood and plasma (liver, bone, and bone marrow actions) | IGF-1, acid-labile subunit | Increased by GH only |
IGF-binding protein-3 | Increased by both GH and IGF-1 | |
Alkaline phosphatase (bone specific) | Increase (mainly IGF-1) | |
Fibrinogen | Increase | |
Hemoglobin, hematocrit | Increase (mainly IGF-1 action on bone marrow) | |
Cartilage, bone | Length (before epiphysial closure), width (periosteal and perichondrial growth) | Stimulation (mainly IGF-1) |
Visceral organs (liver, spleen, thymus, thyroid), tongue and heart | Growth | Stimulation, organomegaly (both GH and IGF-1) |
Renal 25-hydroxyvitamin D 1α-hydroxylase activity | Plasma calcitriol | Increase (mainly GH), promotes positive calcium balance |
Kidney | GFR | Increase (IGF-1) |
Skin | Hair growth | Stimulation (IGF-1?) |
Sweat glands | Hyperplasia, hypertrophy, hyperfunction (GH?) | |
Dermis | Thickening (both GH and IGF-1) |
GH, via IGF-I, increases protein synthesis by enhancing amino acid uptake and directly accelerating the transcription and translation of mRNA. In addition, GH tends to decrease protein catabolism by mobilizing fat as a more efficient fuel source: it directly causes the release of fatty acids from adipose tissue and enhances their conversion to acetyl-CoA, from which energy is derived. This protein-sparing effect is an important mechanism by which GH promotes growth and development.
GH also affects carbohydrate metabolism. In excess, it decreases carbohydrate utilization and impairs glucose uptake into cells. This GH-induced insulin resistance appears to be due to a postreceptor impairment in insulin action. These events result in glucose intolerance and secondary hyperinsulinism.
GH has a plasma half-life of 10 to 20 minutes. The healthy adult secretes approximately 400 μ g/d (18.6 nmol/d); in contrast, young adolescents secrete about 700 μ g/d (32.5 nmol/d). The early morning GH concentration in fasting unstressed adults is less than 2 ng/mL (90 pmol/L). There are no significant sex differences.
About half of circulating GH is bound to specific GH-binding proteins (GHBPs) that function to reduce oscillations in GH levels (due to its pulsatile secretion) and prolong plasma GH half-life. GHBPs include a high-affinity GHBP (corresponding to the extracellular portion of the GH receptor formed through proteolytic cleavage) and a low-affinity species. Measurement of serum concentrations of the high-affinity GHBP provides an index of GH receptor concentrations. For example, individuals with Laron dwarfism, a form of GH insensitivity characterized by mutations in the GH receptor, usually have abnormally low levels of GHBP.
Concentrations of IGF-I are determined by radioreceptor assays or radioimmunoassays. Determining the levels of these mediators of GH action may result in more accurate assessment of the biologic activity of GH (see Chapter 6).
The secretion of GH is predominantly mediated by two hypothalamic hormones: GHRH and somatostatin (GH-inhibiting hormone), both of which contribute to the episodic pattern of GH secretion. These hypothalamic influences are tightly regulated by an integrated system of neural, metabolic, and hormonal factors. Table 4–7 summarizes the many factors that affect GH secretion in physiologic, pharmacologic, and pathologic states.
Increase | Decreasea |
---|---|
Physiologic Sleep Exercise Stress (physical or psychologic) Postprandial
| Postprandial hyperglycemia Elevated free fatty acids |
Pharmacologic Hypoglycemia
Hormones
Neurotransmitters, etc
| Hormones
Neurotransmitters, etc
|
Pathologic Protein depletion and starvation Anorexia nervosa Ectopic production of GHRH Chronic renal failure Acromegaly
| Obesity Acromegaly; dopamine agonists Hypothyroidism Hyperthyroidism |
GHRH binds to specific receptors, stimulating cAMP production by somatotrophs and stimulating both GH synthesis and secretion. The effects of GHRH are partially blocked by somatostatin. The administration of GHRH to normal humans leads to rapid release of GH (within minutes); levels peak at 30 minutes and are sustained for 60 to 120 minutes.
Other peptide hormones such as ADH, ACTH, and α-MSH may act as GH-releasing factors when present in pharmacologic amounts. Even TSH and GnRH often cause GH secretion in patients with acromegaly; however, it is not certain whether any of these effects are mediated by the hypothalamus or represent direct effects on the somatotroph. Regulation of GHRH is primarily under neural control (see discussion later), but there is also short-loop negative feedback by GHRH itself.
Somatostatin, a tetradecapeptide, is a potent inhibitor of GH secretion. It decreases cAMP production in GH-secreting cells and inhibits both basal and stimulated GH secretion. Somatostatin secretion is increased by elevated levels of GH and IGF-I. Long-acting analogs of somatostatin have been used therapeutically in the management of GH excess and in conditions such as pancreatic and carcinoid tumors that cause diarrhea.
Non-GHRH secretagogues act to release GH, not through the GHRH receptor, but through a separate receptor, the growth hormone secretagogue receptor (GHS-R). A number of synthetic secretagogues, both peptides and nonpeptides, have been described. Ghrelin, a circulating peptide made by endocrine cells in the stomach, was identified in 1999 as the endogenous ligand for GHS-R. Its location in the stomach suggests a new mechanism for regulation of GH secretion.
The neural control of basal GH secretion results in irregular and intermittent release associated with sleep and varying with age. Peak levels occur 1 to 4 hours after the onset of sleep (during stages 3 and 4) (Figure 4–13). These nocturnal sleep bursts, which account for nearly 70% of daily GH secretion, are greater in children and tend to decrease with age. Glucose infusion does not suppress this episodic release. Emotional, physical, and chemical stress, including surgery, trauma, exercise, electroshock therapy, and pyrogen administration, provoke GH release. In addition, impairment of secretion leading to growth failure has been well documented in children with severe emotional deprivation (see Chapter 6).
Figure 4–13
Sleep-associated changes in prolactin (PRL) and growth hormone (GH) secretion in humans. Peak levels of GH occur during sleep stages 3 or 4; the increase in PRL is observed 1 to 2 hours after sleep begins and is not associated with a specific sleep phase.
(Reproduced, with permission, from Sassin JF, et al. Human prolactin: 24-hour pattern with increased release during sleep. Science. 1972;177:1205).
The metabolic factors affecting GH secretion include all fuel substrates: carbohydrate, protein, and fat. Glucose administration, orally or intravenously, lowers GH in healthy subjects and provides a simple physiologic maneuver useful in the diagnosis of acromegaly (see discussed below). In contrast, hypoglycemia stimulates GH release. This effect depends on intracellular glycopenia, because the administration of 2-deoxyglucose (a glucose analog that causes intracellular glucose deficiency) also increases GH. This response to hypoglycemia depends on both the rate of change in blood glucose and the absolute level attained.
A protein meal or intravenous infusion of amino acids (eg, arginine) causes GH release. Paradoxically, states of protein-calorie malnutrition also increase GH, possibly as a result of decreased IGF-I production and lack of inhibitory feedback.
Fatty acids suppress GH responses to certain stimuli, including arginine and hypoglycemia. Fasting stimulates GH secretion, possibly as a means of mobilizing fat as an energy source and preventing protein loss.
Responses to stimuli are blunted in states of cortisol excess and during hypo- and hyperthyroidism. Estrogen enhances GH secretion in response to stimulation.
Many neurotransmitters and neuropharmacologic agents affect GH secretion. Biogenic amine agonists and antagonists act at the hypothalamic level and alter GHRH or somatostatin release. Dopaminergic, alpha-adrenergic, and serotonergic agents all stimulate GH release.
Dopamine agonists such as levodopa, apomorphine, and bromocriptine increase GH secretion, whereas dopaminergic antagonists such as phenothiazines inhibit GH. The effect of levodopa, a precursor of both norepinephrine and dopamine, may be mediated by its conversion to norepinephrine, because its effect is blocked by the alpha-adrenergic antagonist phentolamine. Moreover, phentolamine suppresses GH release in response to other stimuli such as hypoglycemia, exercise, and arginine, emphasizing the importance of alpha-adrenergic mechanisms in modulating GH secretion.
Beta-adrenergic agonists inhibit GH, and beta-adrenergic antagonists such as propranolol enhance secretion in response to provocative stimuli.
PRL is a 198-amino-acid polypeptide hormone (MW 22,000) synthesized and secreted from the lactotrophs of the anterior pituitary. Despite evolution from an ancestral hormone common to GH and human placental lactogen (hPL), PRL shares only 16% of its residues with the former and 13% with hPL. A precursor molecule (MW 40,000-50,000) is also secreted and may constitute 8% to 20% of the PRL plasma immunoreactivity in healthy persons and in patients with PRL-secreting pituitary tumors. PRL and GH are structurally related to members of the cytokine-hematopoietin family that include erythropoietin, granulocyte-macrophage colony stimulating factor (GM-CSF), and interleukins IL-2 to IL-7.
PRL stimulates lactation in the postpartum period (see Chapter 16). During pregnancy, PRL secretion increases and, in concert with many other hormones (estrogen, progesterone, hPL, insulin, and cortisol), promotes additional breast development in preparation for milk production. Despite its importance during pregnancy, PRL has not been demonstrated to play a role in the development of normal breast tissue in humans. During pregnancy, estrogen enhances breast development but blunts the effect of PRL on lactation; the decrease in both estrogen and progesterone after parturition allows initiation of lactation. Accordingly, galactorrhea may accompany the discontinuance of oral contraceptives or estrogen therapy. Although basal PRL secretion falls in the postpartum period, lactation is maintained by persistent breast suckling.
PRL levels are very high in the fetus and in newborn infants, declining during the first few months of life.
Although PRL does not appear to play a physiologic role in the regulation of gonadal function, hyperprolactinemia in humans leads to hypogonadism. In women, initially there is a shortening of the luteal phase; subsequently, anovulation, oligomenorrhea or amenorrhea, and infertility occur. In men, PRL excess leads to decreased testosterone synthesis and decreased spermatogenesis, which clinically present as decreased libido, impotence, and infertility. The exact mechanisms of PRL inhibition of gonadal function are unclear, but the principal one appears to be alteration of hypothalamic-pituitary control of gonadotropin secretion. Basal LH and FSH levels are usually normal; however, their pulsatile secretion is decreased and the midcycle LH surge is suppressed in women. Gonadotropin reserve, as assessed by administration of exogenous GnRH, is usually normal. PRL also has a role in immunomodulation; extrapituitary synthesis of PRL occurs in T lymphocytes (among other sites), and PRL receptors are present on T and B lymphocytes and macrophages. PRL modulates and stimulates both immune cell proliferation and survival.
The PRL secretory rate is approximately 400 μ g/d (18.6 nmol/d). The hormone is cleared by the liver (75%) and the kidney (25%), and its half-time of disappearance from plasma is about 25 to 50 minutes.
Basal levels of PRL in adults vary considerably, with a mean of 13 ng/mL (0.6 nmol/L) in women and 5 ng/mL (0.23 nmol/L) in men. The upper range of normal in most laboratories is 15 to 20 ng/mL (0.7-0.9 nmol/L).
PRL is measured using a highly specific immunoradiometric assay. However, when PRL levels are extremely high, which can occur with some PRL-secreting tumors, this assay may be susceptible to the “hook effect.” PRL levels are erroneously reported as normal or modestly elevated due to saturation of available assay antibodies. Appropriate sample dilutions (ie, 1:100) will avoid this artifact.
In some patients a form of PRL with molecular mass greater than 150 kDa predominates. This is termed macroprolactinemia and consists of aggregates of monomeric PRL as well as PRL-immunoglobulin G complexes. These complexes may have reduced biological activity and can be measured using polyethylene glycol precipitation of serum samples.
The hypothalamic control of PRL secretion is predominantly inhibitory, and dopamine is the most important inhibitory factor. The physiologic, pathologic, and pharmacologic factors influencing PRL secretion are listed in Table 4–8.
Increase | Decrease |
---|---|
Physiologic Pregnancy Nursing Nipple stimulation Exercise Stress (hypoglycemia) Sleep Seizures Neonatal | |
Pharmacologic TRH Estrogen Vasoactive intestinal peptide Dopamine antagonists (phenothiazines, haloperidol, risperidone, metoclopramide, reserpine, methyldopa, amoxapine, opioids) Monoamine oxidase inhibitors Cimetidine (intravenous) Verapamil Licorice | Dopamine agonists (levodopa, apomorphine, bromocriptine, pergolide) GABA |
Pathologic Pituitary tumors Hypothalamic/pituitary stalk lesions Neuraxis irradiation Chest wall lesions Spinal cord lesions Hypothyroidism Chronic renal failure Severe liver disease | Pseudohypoparathyroidism Pituitary destruction or removal Lymphocytic hypophysitis |
TRH is a potent PRL-releasing factor that evokes release of PRL at a threshold dose similar to that which stimulates release of TSH. An exaggerated response of both TSH and PRL to TRH is observed in primary hypothyroidism, and their responses are blunted in hyperthyroidism. In addition, PRL secretion is also stimulated by VIP and serotonergic pathways.
PRL secretion is episodic. An increase is observed 60 to 90 minutes after sleep begins but—in contrast to GH—is not associated with a specific sleep phase. Peak levels are usually attained between 4 and 7 AM (see Figure 4–13). This sleep-associated augmentation of PRL release is not part of a circadian rhythm, like that of ACTH; it is related strictly to the sleeping period regardless of when it occurs during the day.
Stresses, including surgery, exercise, hypoglycemia, and acute myocardial infarction, cause significant elevation of PRL levels. Nipple stimulation in nonpregnant women also increases PRL. This neurogenic reflex may also occur from chest wall injury such as mechanical trauma, burns, surgery, and herpes zoster of thoracic dermatomes. This reflex discharge of PRL is abolished by denervation of the nipple or by spinal cord or brain stem lesions.
Many hormones influence PRL release. Estrogens augment basal and stimulated PRL secretion after 2 to 3 days of use (an effect that is of special clinical importance in patients with PRL-secreting pituitary adenomas); glucocorticoids tend to suppress TRH-induced PRL secretion; and thyroid hormone administration may blunt the PRL response to TRH.
Many pharmacologic agents alter PRL secretion. Dopamine agonists (eg, bromocriptine) decrease secretion, forming the basis for their use in states of PRL excess. Dopamine antagonists (eg, receptor blockers such as phenothiazines and metoclopramide) and dopamine depletors (eg, reserpine) augment PRL release. Serotonin agonists enhance PRL secretion; serotonin receptor blockers suppress PRL release associated with stress and with nursing.
TSH is a glycoprotein (MW 28,000) composed of two noncovalently linked alpha and beta subunits. The structure of the alpha subunit of TSH is identical to that of the other glycoprotein molecules—FSH, LH, and human chorionic gonadotropin (hCG)—but the beta subunits differ in these glycoproteins and is responsible for their biologic and immunologic specificity. The peptides of these subunits appear to be synthesized separately and united before the carbohydrate groups are attached. The intact molecule is then secreted, as are small amounts of nonlinked subunits.
The beta subunit of TSH attaches to high-affinity receptors in the thyroid, stimulating iodide uptake, hormonogenesis, and release of T4 and T3. This occurs through activation of adenylyl cyclase and the generation of cAMP. TSH secretion also causes an increase in gland size and vascularity by promoting mRNA and protein synthesis. (For a more detailed description, see Chapter 7.)
TSH circulates unbound in the blood with a half-life of 35 to 50 minutes. With ultrasensitive IRMAs for measuring TSH concentration, the normal range is usually 0.5 to 4.7 μ U/mL (0.5-4.7 mU/L). These new assays are helpful in the diagnosis of primary hypothyroidism and hyperthyroidism; however, TSH levels alone cannot be used to evaluate pituitary or hypothalamic hypothyroidism.
The alpha subunit can be detected in about 80% of normals, with a range of 0.5 to 2 ng/mL. Plasma alpha subunit levels increase after administration of TRH in normal subjects, and basal levels are elevated in primary hypothyroidism, primary hypogonadism, and in patients with TSH-secreting, gonadotropin-secreting, or pure alpha subunit-secreting pituitary adenomas.
The secretion of TSH is controlled by both stimulatory (TRH) and inhibitory (somatostatin) influences from the hypothalamus and in addition is modulated by the feedback inhibition of thyroid hormone on the hypothalamic-pituitary axis.
The response of TSH to TRH is modulated by the circulating concentration of thyroid hormones. Small changes in serum levels (even within the physiologic range) cause substantial alterations in the TSH response to TRH. As shown in Figure 4–14, the administration of T3 (15 μ g) and T4 (60 μ g) to healthy persons for 3 to 4 weeks suppresses the TSH response to TRH despite only small increases in circulating T3 and T4 levels. Thus, the secretion of TSH is inversely proportional to the concentration of thyroid hormone.
Figure 4–14
Administration of small doses of T3 (15 μg) and T4 (60 μg) to healthy subjects inhibits the TSH response to two doses (400 μg, left; 25 μg, right) of TRH (protirelin).
(Reproduced, with permission, from Snyder PJ, Utiger RD. Inhibition of thyrotropin-releasing hormone by small quantities of thyroid hormones. J Clin Invest. 1972;51:2077).
The set point (the level at which TSH secretion is maintained) is determined by TRH. Deviations from this set point result in appropriate changes in TSH release. Administration of TRH increases TSH within 2 minutes, and this response is blocked by previous T3 administration; however, larger doses of TRH may overcome this blockade—suggesting that both T3 and TRH act at the pituitary level to influence TSH secretion. In addition, T3 and T4 inhibit mRNA for TRH synthesis in the hypothalamus, indicating that a negative feedback mechanism operates at this level also.
This inhibitory hypothalamic peptide augments the direct inhibitory effect of thyroid hormone on the thyrotrophs. Infusion of somatostatin blunts the early morning TSH surge and suppresses high levels of TSH in primary hypothyroidism. Octreotide acetate, a somatostatin analog, has been used successfully to inhibit TSH secretion in patients with TSH-secreting pituitary tumors.
In addition to these hypothalamic influences on TSH secretion, neurally mediated factors may be important. Dopamine physiologically inhibits TSH secretion. Intravenous dopamine administration decreases TSH in both healthy and hypothyroid subjects as well as blunts the TSH response to TRH. Thus, as expected, dopaminergic agonists such as bromocriptine inhibit TSH secretion and dopaminergic antagonists such as metoclopramide increase TSH secretion in euthyroid subjects.
Glucocorticoid excess has been shown to impair the sensitivity of the pituitary to TRH and may lower serum TSH to undetectable levels. However, estrogens increase the sensitivity of the thyrotroph to TRH; women have a greater TSH response to TRH than men do, and pretreatment of men with estradiol increases their TRH-induced TSH response. (See also Chapter 7.)
LH and FSH are glycoprotein gonadotropins composed of alpha and beta subunits and secreted by the same cell. The specific beta subunit confers on these hormones their unique biologic activity, as it does with TSH and hCG. The biologic activity of hCG, a placental glycoprotein, closely resembles that of LH. Human menopausal gonadotropin (hMG, menotropins)—an altered mixture of pituitary gonadotropins recovered from the urine of postmenopausal women—is a preparation with FSH-like activity. Menotropins and hCG are used clinically for induction of spermatogenesis or ovulation (see Chapters 12 and 13).
LH and FSH bind to receptors in the ovary and testis and regulate gonadal function by promoting sex steroid production and gametogenesis.
In men, LH stimulates testosterone production from the interstitial cells of the testes (Leydig cells). Maturation of spermatozoa, however, requires both LH and FSH. FSH stimulates testicular growth and enhances the production of an androgen-binding protein by the Sertoli cells, which are a component of the testicular tubule necessary for sustaining the maturing sperm cell. This androgen-binding protein promotes high local concentrations of testosterone within the testis, an essential factor in the development of normal spermatogenesis (see Chapter 12).
In women, LH stimulates estrogen and progesterone production from the ovary. A surge of LH in the midmenstrual cycle is responsible for ovulation, and continued LH secretion subsequently stimulates the corpus luteum to produce progesterone by enhancing the conversion of cholesterol to pregnenolone. Development of the ovarian follicle is largely under FSH control, and the secretion of estrogen from this follicle is dependent on both FSH and LH.
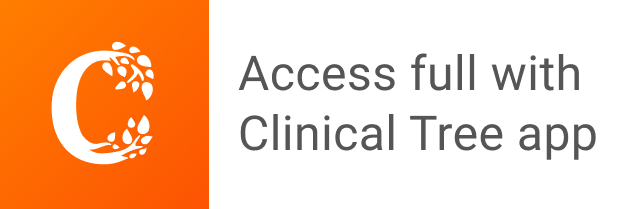