Abbreviations
- ACTH Adrenocorticotropin
- AD1 Activation domain 1
- AD2 Activation domain 2
- AF-1 Activator function-1
- AF-2 Activator function-2
- Akt Protein kinase B AMH Anti-müllerian hormone
- ANP Atrial natriuretic peptide
- AP-1 Activator protein-1
- β-ARK β-Adrenergic receptor kinase
- BNP B-type natriuretic peptide
- cAMP Cyclic adenosine-3′,5′-monophosphate
- CARM Coactivator-associated arginine methyltransferase
- CBP CREB-binding protein
- cGMP Cyclic guanosine-3′,5′-monophosphate
- CNP C-type natriuretic peptide
- CREB cAMP response element-binding protein
- DAG Diacylglycerol
- DBD DNA-binding domain
- DRIP Vitamin D receptor–interacting protein
- EGF Epidermal growth factor
- ER Estrogen receptor
- ERK Extracellular signal–regulated kinase
- FAD Flavin adenine dinucleotide
- FGF Fibroblast growth factor
- FMN Flavin mononucleotide
- FOX A1 Forkhead transcription factor A1
- GAP GTPase-activating protein
- GAS Interferon gamma activated sequences
- GDP Guanosine diphosphate GH Growth hormone GHR Growth hormone receptor
- GLUT 4 Glucose transporter type 4
- GR Glucocorticoid receptor
- GRB2 Growth factor receptor–bound protein-2 GRE Glucocorticoid response element
- GRIP Glucocorticoid receptor–interacting protein
- GSK3 Glycogen synthase kinase-3
- GTF General transcription factor
- GTP Guanosine triphosphate
- HRE Hormone response element
- HSP Heat shock protein
- ID Receptor–repressor interaction domain
- IGF Insulin-like growth factor
- I-κB Inhibitor of nuclear factor kappa B
- IKK Inhibitor of nuclear factor kappa B kinase
- IP3 Inositol 1,4,5-trisphosphate
- IP4 Inositol 1,3,4,5-tetrakis-phosphate
- ISRE Interferon-stimulated response element
- JAK Janus kinase
- KHD Kinase homology domain
- LBD Ligand-binding domain
- LH Luteinizing hormone
- MAPK Mitogen-activated protein kinase
- MEK MAPK kinase
- MR Mineralocorticoid receptor
- MSH Melanocyte-stimulating hormone
- N-Cor Nuclear receptor corepressor
- NF-κB Nuclear factor kappa B NO Nitric oxide NOS Nitric oxide synthase
- NPR Natriuretic peptide receptor
- NR Nuclear receptor
- NRPTK Nonreceptor protein tyrosine kinase
- PAK p21-activated kinase
- P/CAF p300/CBP-associated factor
- P/CIP p300/CBP cointegrator-associated protein
- PDE Phosphodiesterase
- PDGF Platelet-derived growth factor
- PDK Phosphatidylinositol-3,4,5- triphosphate-dependent kinase
- PHP-1a Pseudohypoparathyroidism type 1a
- PI-3K Phosphoinositide-3-OH kinase
- PIP2 Phosphatidylinositol-4,5-bisphosphate
- PIP3 Phosphatidylinositol-3,4,5-trisphosphate
- PI(3,4)P2 Phosphatidylinositol-3,4,5-bisphosphate
- PKA Protein kinase A
- PKB Protein kinase B
- PKC Protein kinase C
- PKG cGMP-dependent protein kinase
- PLCβ Phospholipase C beta
- PLCγ Phospholipase C gamma
- PLCPC Phosphatidylcholine-selective phospholipase
- POL II RNA polymerase II
- PPARγ Peroxisome proliferator–activated receptor
- PR Progesterone receptor
- PTH Parathyroid hormone
- RANK Receptor activator of nuclear factor kappa B
- RAR Retinoic acid receptor
- RE Response element
- RGS Regulators of G protein signaling
- RSK Ribosomal S6 kinase
- RXR Retinoid X receptor
- SH2 src homology domain type 2
- SIE Sis-inducible element
- SMRT Silencing mediator for RXR and TR
- SOCS Suppressor of cytokine signaling
- SOS Son-of-sevenless
- SR Steroid receptor
- SRC Steroid receptor coactivator
- SRE Serum response element
- SRF Serum response factor
- STAT Signal transducer and activator of transcription
- SWI/SNF ATP-dependent chromatin remodeling complex
- TBP TATA-binding protein
- TGF-β Transforming growth factor beta
- TPA 12-O-tetradecanoyl-phorbol 13-acetate
- TR Thyroid hormone receptor
- TRAF Tumor necrosis factor receptor–associated factor
- TRAP Thyroid hormone receptor–associated protein
- TRE TPA response element
- TSH Thyroid-stimulating hormone
- VDR Vitamin D receptor
Hormones and Hormone Action: Introduction
Hormones are signaling molecules that traffic information from one cell to another, typically through a soluble medium like the extracellular fluid. Hormones fall into one of a number of different hormonal classes (eg, steroids, monoamines, peptides, proteins, eicosanoids) and signal through a variety of general (eg, nuclear vs cell surface) and specific (eg, tyrosine kinase vs phosphoinositide turnover) mechanisms in target cells.
Hormones produced in one tissue may promote activity in a target tissue at some distance from the point of secretion. In this case the hormone travels through the bloodstream, often bound to a plasma protein, to access the target tissue. In addition, hormones may act locally following secretion; either on a neighboring cell (paracrine effect), on the secretory cell itself (autocrine effect), or without actually being released from the secretory cell (intracrine effect) (Figure 1–1).
Figure 1–1
Actions of hormones and neurotransmitters. Endocrine and neurotransmitter cells synthesize hormones and release them by specialized secretory pathways or by diffusion. Hormones can act at the site of production either following release (autocrine) or without release (intracrine) from the producer cell. They can also act on neighboring target cells, including neurotransmitter-producing cells, without entering the circulation (paracrine). Finally, they can access target cells through the circulation (hormonal). Neurotransmitters that access the extracellular compartment, including circulating plasma, can act as paracrine or hormonal regulators of target cell activity (H, hormone; N, neurotransmitter; R, receptor).
Relationship to the Nervous System
Many features of the endocrine system, for example, the use of ligands and receptors to establish communication between cells, are shared by the nervous system. In fact, from a functional standpoint, the two systems are probably related from an evolutionary standpoint. However, there are some important differences between the two systems. While the nervous system uses a highly compartmentalized, closed system of cables to connect cells at some distance from one another, the endocrine system relies on circulating plasma to carry newly released hormone to its targets in the periphery. As a result, the time constants for signal delivery are quite different between the two—virtually instantaneous for the nervous system but delayed, by virtue of circulation times, for the endocrine system. Thus, while neural responses are typically measured in seconds, endocrine responses are measured in minutes to hours—thereby accommodating different needs in the organism. A second difference relates to the nature of the ligand–receptor interaction. In the nervous system, the affinity of receptor for ligand is relatively low. This allows for rapid dissociation of ligand from receptor and, if that ligand is degraded locally, a rapid cessation of biological effect. Despite this rapid dissociation, the secretory neuron is able to maintain receptor occupancy by keeping concentrations of the ligand high around the target neuron. It does this through pulsatile release of secretory granules into an incredibly small volume (ie, that determined by the volume in the synaptic cleft). The endocrine system, on the other hand, has a very large volume of distribution for many of its ligands (eg, circulating blood volume). Maintaining ligand concentrations analogous to those present in the synaptic cleft would require prodigious secretory capacity. The endocrine system circumvents this problem by selecting ligand–receptor interactions of much higher affinity (100-10,000 fold higher binding affinity) than those used in the nervous system. In effect, the nervous system is structured to deliver high ligand concentrations to relatively low-affinity receptors, allowing it to activate and inactivate biological effects quickly and in a relatively well-defined topography. Its effects are short lived. The endocrine system, on the other hand, uses high-affinity receptors to extract and retain ligand from a relatively “dilute” pool in circulating plasma. Its biological effects are longlasting. It has sacrificed the rapid response to accommodate a wider area of signal distribution and prolongation of the biological effect. Thus, the systems are not only related but complementary in the respective roles that they play in contributing to normal physiological function.
Chemical Nature of Hormones
Hormones vary widely in terms of their chemical composition. Specific examples include proteins (eg, adrenocorticotrophin), peptides (eg, vasopressin), monoamines (eg, norepinephrine), amino acid derivatives (eg, triiodothyronine), steroids (eg, cortisol), and lipids (eg, prostaglandins). Proteins can be glycosylated (eg, thyroid-stimulating hormone) and/or dimerized (eg, follicle-stimulating hormone) to generate full biological activity. In general, protein, peptide, monoamine, and lipophilic hormones tend to exert their effects primarily through protein receptors at the cell membrane, while thyroid hormone and steroids tend to operate in the cell nucleus. However, there are increasing numbers of exceptions to these rules (eg, triiodothyronine activates classic thyroid hormone receptors in the nuclear compartment, and the trace amine receptor [TAR1]) on the cell surface and estradiol appears to activate both nuclear and plasma membrane receptors). It is likely that the biological “effect” of a given hormone reflects a composite of receptor activity located in several different cellular compartments.
Endocrine Glands and Target Organs
Endocrine glands are traditionally defined as the ductless glandular structures that release their hormonal secretions into the extracellular space where they eventually access circulating plasma. Classic endocrine glands include organs like the pituitary gland, thyroid gland, parathyroid glands, pancreatic islets, adrenal glands, ovaries, and testes. It is now clear that hormones can be secreted from nontraditional endocrine organs and play critical roles in the regulation of physiological homeostasis. Examples of the latter include the heart (natriuretic peptides), kidney (erythropoietin and renin), adipose tissue (leptin and adiponectin), and gut (cholecystokinin and incretins). Once in the circulation, hormones bind to receptors on target tissues to elicit their biological effects. Target tissues for some hormones (eg, glucocorticoids) are numerous reflecting the ubiquitous distribution of glucocorticoid receptors, while those for other tissues have a more limited distribution (eg, androgens).
Regulation of Hormone Levels in Plasma
Hormone levels in plasma determine the effective ligand concentration at the level of the hormone receptors in peripheral target cells. Thus, regulation of hormone levels plays an important role in the control of the biological effects that the hormone exerts.
New hormone synthesis is one of the principal mechanisms used to raise hormone levels in circulating plasma. In the case of protein or peptide hormones this usually reflects increased expression of the gene encoding the hormone (ie, increased production of the mRNA encoding the hormone) with subsequent increase in hormone synthesis. In the case of steroid or thyroid hormones it reflects increased sequestration of precursors for hormone synthesis (eg, cholesterol for steroid hormones or iodide for thyroid hormone) as well as increased activity of those enzymatic proteins responsible for executing the individual catalytic events required for hormone production—typically the latter may involve a rate-limiting step in the synthetic cascade (eg, 1-alpha hydroxylase activity in the synthesis of 1,25- dihydroxyvitamin D).
Processing of hormone precursors participates to varying degrees in controlling circulating hormone levels. Most peptide and protein hormones require some degree of processing to generate the mature hormonal product (eg, conversion of proinsulin to insulin) and impairment in the processing activity can alter the ratio of precursor to product in plasma. In other cases, a critical processing event is part of the secretory process itself (eg, cleavage of thyroxine from thyroglobulin) and impaired processing can result in a dramatic reduction in immunoreactivity as well as bioactivity of the mature hormone. In addition, protein hormones may require posttranslational modification (eg, glycosylation) or assembly (eg, heterodimerization) prior to secretion in order to optimize biological activity.
Many hormones (eg, peptides, proteins, and monoamines) are stored in secretory granules in endocrine cells. Release of these granules is promoted by signaling events triggered by exogenous regulators termed secretagogues. This often requires activation of a second messenger system (see discussion under “Receptors”) like cyclic AMP generation or intracellular calcium mobilization in the endocrine cell. Steroid hormones, on the other hand, are not stored to a significant degree in the hormone-producing cells. In this case synthesis rather than hormone release appears to play the dominant role in controlling hormone levels in circulating plasma.
Hormones in plasma can circulate either in a free form, uncomplexed with other molecules, or bound to other molecules like plasma proteins. It is the uncomplexed or free form of the hormone that represents the biologically active fraction of hormone in the plasma compartment, and it is this fraction which homeostatic regulatory mechanisms work to preserve. However, binding of hormone to plasma proteins does play an important role in endocrine physiology. First, it provides a reservoir of hormone that exchanges with the free hormone fraction according to the laws of mass action (see under “Receptors”). This makes plasma hormone concentrations less dependent on hormone synthesis and release, effectively stabilizing those concentrations over extended periods of time. This also serves to guarantee a uniform distribution of hormone concentration in capillary beds perfusing target tissues (Figure 1–2). Second, it slows the metabolism or turnover of the hormone by sequestering it away from degradative enzymes or filtration by the kidney.
Figure 1–2
Role of plasma binding in delivery of hormones to peripheral tissues. Example shows a hormone that is bound (small red circles) to a plasma protein (large circles) and a hormone that is not protein bound (small orange circles). With the bound hormone, only the free fraction is available for tissue uptake. As the free fraction is depleted, additional hormone dissociates from the plasma-binding protein, making hormone available to more distal portions of the tissue. In contrast, all hormones that are not protein bound are quickly extracted in the proximal part of the tissue.
Metabolism of hormones also plays an important role in regulating hormone concentrations. In some cases metabolism is responsible for converting precursors with less hormonal activity to products with greater activity (eg, conversion of 25-hydroxyvitamin D to 1,25-dihydroxyvitamin D and conversion of androstenedione to testosterone). In other cases, metabolism leads to degradation and inactivation of the hormone with a cessation of hormone activity. This type of degradation is often specific to the hormonal class under examination. Steroids, for example, are catalytically converted to inactive metabolites and/or sulfated to promote excretion. Thyroid hormones are subjected to deiodination which strips them of their biological activity. Protein and peptide hormones are internalized by target, as well as nontarget, cells and degraded in intracellular lysosomes. In general, the more avid the degradative mechanisms, the shorter the plasma half-life of the hormone.
Hormone levels can be modulated through regulatory factors affecting any of the steps listed earlier; however, the bulk of the acute “fine-tuning” of hormone levels occurs at the level of hormone secretion and synthesis. Many, if not most, hormone levels are controlled either directly or indirectly by the biological activity that they serve to control. For example, parathyroid hormone (PTH) secretion which responds to low extracellular calcium levels, mobilizes calcium out of bone which, in turn, signals back to the parathyroid gland to turn off PTH secretion. This negative feedback inhibition is a hallmark of endocrine regulation. The end product or negative regulator can either be an inorganic ion or metabolite (eg, calcium for PTH) or a hormonal product in the endocrine cascade (eg, thyroid hormone for TSH). Not all feedback is negative in nature and positive feedback loops (eg, mid-cycle estradiol-induced luteinizing hormone secretion) also play important roles in governing physiological homeostasis.
Hormone Action
Hormones produce their biologic effects through interaction with high-affinity receptors that are, in turn, linked to one or more effector systems within the cell. These effectors involve many different components of the cell’s metabolic machinery, ranging from ion transport at the cell surface to stimulation of the nuclear transcriptional apparatus. Steroids and thyroid hormones exert their effects in the cell nucleus, although regulatory activity in the extranuclear compartment has also been documented. Peptide hormones and neurotransmitters, on the other hand, trigger a plethora of signaling activity in the cytoplasmic and membrane compartments while at the same time exerting parallel effects on the transcriptional apparatus. The discussion that follows will focus on the primary signaling systems employed by selected hormonal agonists and attempt to identify examples where aberrant signaling results in human disease.
Receptors
The biologic activity of individual hormones is dependent on their interactions with specific high-affinity receptors on the surfaces or in the cytoplasm or nuclei of target cells. The receptors, in turn, are linked to signaling effector systems responsible for generating the observed biologic response. Receptors, therefore, convey not only specificity of the response (ie, cells lacking receptors lack responsiveness to the hormone) but also the means for activating the effector mechanism. In general, receptors for the peptide hormones and neurotransmitters are aligned on the cell surface and those for the steroid hormones, thyroid hormone, and vitamin D are found in the cytoplasmic or nuclear compartments.
where [H] is the hormone concentration, [R] is the receptor concentration, [HR] is the concentration of the hormone–receptor complex, and k+1 and k−1 are the rate constants for [HR] formation and dissociation, respectively. Thus, at equilibrium,
where KD is the equilibrium dissociation constant that defines the affinity of the hormone–receptor interaction (ie, lower the dissociation constant, higher the affinity). Assuming that total receptor concentration R0 = [HR] + [R], this equation can be rearranged to give
This is the Scatchard equation and states that when bound over free ligand (ie, [HR]/[H]) is plotted against bound ligand (ie, [HR]), the slope of the line is defined by 1/KD, the y-intercept by R0/KD, and the x-intercept by R0 (Figure 1–3). When [HR] = R0/2, [H] = KD; therefore, the KD is also the concentration of hormone [H] at which one-half of the available receptors are occupied. Thus, knowledge of bound and free ligand concentrations, which can be determined experimentally, provides information regarding the affinity of the receptor for its ligand and the total concentration of receptor in the preparation.
Figure 1–3
Ligand saturation (A) and Scatchard analysis (B) of a hypothetical hormone receptor interaction. KD represents the dissociation constant; R0 the total receptor concentration; [HR] and [H] the bound and free ligand, respectively. Note in A that the KD is the concentration [H] at which half of available receptors are occupied.
Agents that bind to receptors with high affinity are classified as either agonists or antagonists based on the functional outcome of this receptor–ligand interaction. Agonists are ligands that trigger the effector mechanisms and produce biologic effects. Antagonists bind to the receptor but do not activate the effector mechanisms. Because they occupy receptor and block association with the agonist, they antagonize the functional activity of the latter. Partial agonists bind to the receptor but possess limited ability to activate the effector mechanisms. In different circumstances, partial agonists demonstrate variable biologic activity. For example, when used alone, they may display weak activating activity, whereas their use together with a full agonist may lead to inhibition of function because the latter is displaced from the receptor molecule by a ligand with lower intrinsic activity.
In some systems, receptors are available in a surplus, which is severalfold higher than that required to elicit a maximal biologic response. Such spare receptor systems, although they superficially appear redundant, are designed to rectify a mismatch between low circulating ligand levels and a relatively low-affinity ligand–receptor interaction. Thus, by increasing the number of available receptors, the system is guaranteed a sufficient number of liganded receptor units to activate downstream effector systems fully, despite operating at subsaturating levels of ligand.
Neurotransmitter and Peptide Hormone Receptors
As mentioned above, neurotransmitter and peptide hormones interact predominantly with receptors expressed on the plasma membrane at the cell surface. The KD of a neurotransmitter for its receptor is typically higher than that of a hormone for its receptor, reflecting a higher koff rate constant (see earlier). Neurotransmitter receptor occupancy is driven by the extraordinarily high concentrations of ligand that can be achieved in the synaptic cleft, and occupancy of the hormone receptor is driven by its high affinity for ligand. The high koff of the neurotransmitter–receptor interaction guarantees that the effect is rapid in onset but of short duration, whereas the lower koff of the hormone–receptor interaction guarantees that the effect is slow in onset but difficult to extinguish, kinetics that are more appropriate for the hormonal functions of these ligands.
The neurotransmitter and peptide receptors can be divided into several major groups (Table 1–1 and Figure 1–4). The first includes the so-called serpentine or “seven-transmembrane-domain” receptors. These receptors each contain an amino terminal extracellular domain followed by seven hydrophobic amino acid segments, each of which is believed to span the membrane bilayer (Figure 1–4). The seventh of these, in turn, is followed by a hydrophilic carboxyl terminal domain that resides within the cytoplasmic compartment. As a group, they share a dependence on the G protein transducers (see discussed later) to execute many of their biologic effects. A second group includes the single-transmembrane-domain receptors that harbor intrinsic tyrosine kinase activity. This includes the insulin, insulin-like growth factor (IGF), and epidermal growth factor (EGF) receptors. A third group, which is functionally similar to the second group, is characterized by a large, extracellular binding domain followed by a single membrane-spanning segment and a cytoplasmic tail. These receptors do not possess intrinsic tyrosine kinase activity but appear to function through interaction with soluble transducer molecules which do possess such activity. Prolactin and growth hormone are included in this group. Another group are the transforming growth factor beta (TGF-β) family members which signal through serine/threonine kinase domains in their cytoplasmic tails. A fifth group, which includes the natriuretic peptide receptors, operates through activation of a particulate guanylyl cyclase and synthesis of cGMP. The cyclase is covalently attached at the carboxyl terminal portion of the ligand-binding domain and thus represents an intrinsic part of the receptor molecule.
Seven-Transmembrane Domain |
β-Adrenergic |
PTH |
LH |
TSH |
GRH |
TRH |
ACTH |
MSH |
Glucagon |
Dopamine |
α2-Adrenergic (−) |
Somatostatin (−) |
Single-Transmembrane Domain |
Growth factor receptors |
Insulin |
IGF |
EGF |
PDGF |
Cytokine receptors |
Growth hormone |
Prolactin |
Erythropoietin |
CSF |
Guanylyl cyclase-linked receptors |
Natriuretic peptides |
G Protein–Coupled Receptors
G protein–coupled receptors constitute a large superfamily of molecules capable of responding to ligands of remarkable structural diversity—ranging from photons to large polypeptide hormones. These receptors share overall structural features, most notably seven membrane-spanning regions connected by intracellular and extracellular loops (see Figure 1–4). The receptors are oriented such that the amino terminal domain is extracellular, whereas the carboxyl terminal tail is cytoplasmic. The membrane-spanning segments interact with one another, forming an irregular cylindrical bundle around a central cavity within the molecule. G protein–coupled receptors can assume at least two conformations with differing orientations of the membrane-spanning segments relative to one another. One orientation is favored in the absence of an agonist ligand, and in this orientation the receptor does not activate a G protein (inactive conformation). The second orientation is stabilized by the binding of an appropriate agonist ligand, and in this conformation the receptor activates a cognate G protein (active conformation). All G protein–coupled receptors are thought to undergo a similar conformational switch on agonist binding, producing a structural change in the cytoplasmic domain that promotes G protein activation. Some small agonists, such as catecholamines, are able to enter the cavity formed by the transmembrane segments, thereby directly stabilizing the active receptor conformation. Other agonists, such as large polypeptide hormones, bind primarily to the extracellular domain of their G protein–coupled receptors. This indirectly results in movement of the transmembrane region of the receptor and stabilization of the active receptor conformation.
Until recently, it was thought that G protein–coupled receptors function exclusively as monomers. Many G protein–coupled receptors are now known to dimerize either with themselves (homodimerization) or with other G protein–coupled receptors (heterodimerization). In some cases, dimerization is important for efficient receptor biosynthesis and membrane localization. In other cases, dimerization is important for optimal ligand affinity, specificity, or receptor signaling.
Heritable mutations in a variety of G protein–coupled receptors are known to be associated with disease. Loss-of-function phenotypes result from mutations that eliminate one or both receptor alleles, or that result in the synthesis of signaling-defective receptors. Gain-of-function phenotypes generally result from point mutations that produce constitutively active receptors (ie, stably assume the active receptor conformation even in the absence of an agonist ligand). Examples of such G protein–coupled receptor disorders relevant to endocrinology are described below and discussed in greater detail elsewhere in this book.
G Protein Transducers
G protein–coupled receptors initiate intracellular signaling by activating one (or in some cases multiple) G proteins. G proteins are a family of heterotrimeric proteins that regulate the activity of effector molecules (eg, enzymes, ion channels) (Table 1–2), resulting ultimately in biological responses. The identity of a G protein is defined by the nature of its α subunit, which is largely responsible for effector activation. The major G proteins involved in hormone action (and their actions on effectors) are Gs (stimulation of adenylyl cyclase), Gi (inhibition of adenylyl cyclase; regulation of calcium and potassium channels), and Gq/11 (stimulation of phospholipase C [PLC] β). The β and γ subunits of G proteins are tightly associated with one another and function as a dimer. In some cases, the βγ subunit dimer also regulates effector function.
G Protein Subunit | Associated Receptors | Effector |
---|---|---|
αs | β-Adrenergic TSH Glucagon | Adenylyl cyclase Ca2+ channels K+ channels |
αi | α2-Adrenergic Muscarinic (type II) | Adenylyl cyclase Ca2+ channels K+ channels |
αq | α1-Adrenergic | PLCβ |
β/α | Adenylyl cyclase (+ or −) PLC Supports βARK-mediated receptor phosphorylation and desensitization |
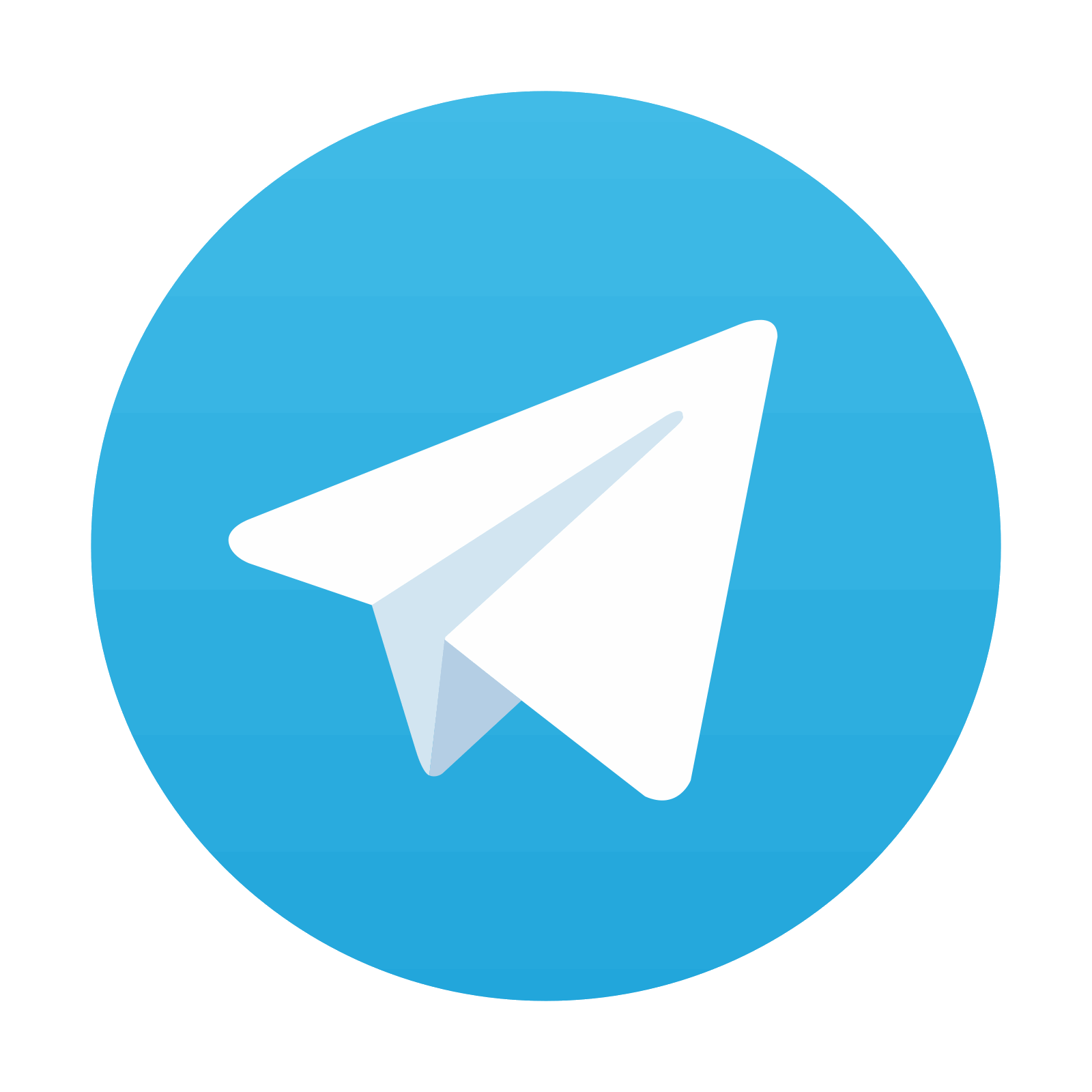
Stay updated, free articles. Join our Telegram channel
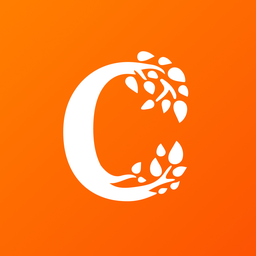
Full access? Get Clinical Tree
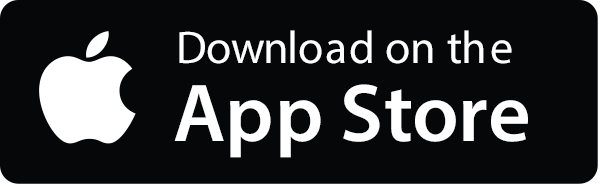
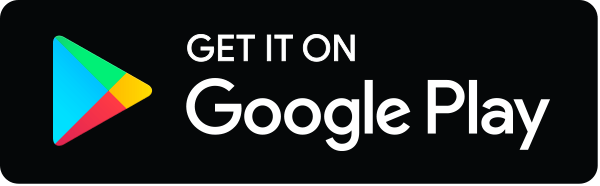