High-intensity focused ultrasound as a trackless, noninvasive method of ablating tissue has been a concept with potential for more than 60 years. It is only in the past 10 to 15 years that technological advances in both the delivery system and the monitoring systems have allowed us to begin to truly explore this potential clinically. Although unique technical challenges remain to be overcome, this ablative technique has been successfully applied in nearly all regions of the body, even some hidden by dense bone. The possibility that HIFU may be synergistic in ways that other ablative therapies are not, with both chemotherapeutics and immune system modifiers, positions HIFU for further investigations into multidisciplinary, individualized therapy unique to the patient.
Thermal ablative therapy has been very successful in broadening the treatment options available in many conditions that are otherwise difficult to treat using purely conventional modalities. Most thermal delivery techniques such as cryotherapy, interstitial laser, electroporation, and radiofrequency require the insertion of an applicator into the target tissue. High-intensity focused ultrasound (HIFU) is an extracorporeal, noninvasive method in which focused ultrasound beams produce complete coagulative necrosis at the target lesions through intact skin without surgical exposure or instrumentation. Lynn and colleagues described the possibility of using ultrasound to carry out destructive surgery of this nature as early as 1942. Because HIFU does not require an internal applicator, there is no risk for puncture-related bleeding or needle track dissemination of malignant cells, and it is effective even when the tumor is poorly perfused (as opposed to electroconductive treatments that require a relatively uniformly hydrated treatment volume). Sound waves enter the body over a broad surface area with diffuse energy insufficient to cause thermal injury, then pass to a single focal point where their combined energy, enhanced by constructive sonic wave interference, is capable of elevating tissue temperature to lethal levels (65°–85°C). It is only in the past 20 years that technology has enabled this concept be safely developed; however, a substantial amount of work remains to refine current technology platforms to allow for treatment throughout the entire body.
The physics of high-intensity focused ultrasound
Sound is vibration. In physics, the term “ultrasound” applies to all acoustic energy (longitudinal, mechanical wave) with a frequency above the audible range of human hearing: between about 20 and 20,000 Hz. Sounds with frequencies higher than this range are termed ultrasound. Planar ultrasound waves deposit clinically insignificant energy as they travel through tissues.
All medical applications of ultrasound make use of the piezoelectric effect ( Fig. 1 A, B). The piezoelectric effect is a reversible process in which certain solid materials (notably crystals and some ceramics) will have internal generation of a mechanical force when an electrical charge is applied (converse piezoelectric effect). These materials will also exhibit the direct piezoelectric effect, namely the generation of an electrical charge when a mechanical force is applied. When a series of electrical pulses (ie, an alternating electrical current) is passed through a piezoelectric crystal, mechanical waves are created that propagate away from the crystal. As the waves pass across an interface of different tissues, the speed of the wave propagation will change. Each time the speed of sound changes, ie, different tissue density, some of it will be reflected, or echoed, back to the crystal, and some will continue propagating forward. When an echo returns, it vibrates the crystal, creating an electric current that can be analyzed and processed to construct an image. Typical diagnostic ultrasound has a single crystal that rapidly alternates between transmission and listening modes. Diagnostic sonographic scanners typically operate in the frequency range of 2 to 18 MHz, the choice of frequency being a trade-off between spatial resolution of the image and imaging depth: lower frequencies produce less resolution but image deeper into the body.
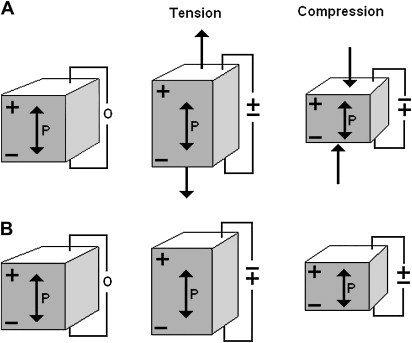
Mechanical index (MI) is the peak rarefaction pressure of an ultrasound longitudinal wave and is a standard measure of the acoustic output in a diagnostic ultrasound system. It is also an important concept to understand how an ultrasonic wave can produce desirable tissue damage. As MI increases, microbubbles are generated within the tissue as the ultrasonic wave physically deforms the tissue. If MI is elevated enough, rupture of these microbubbles will occur. Diagnostic ultrasound becomes therapeutic ultrasound when the peak MI, intended to occur at the focal zone of the ultrasound beam, nears or exceeds this threshold. For diagnostic imaging, intermediate acoustic power (0.1 <MI <0.5) is used, which deposits very little, nondisruptive energy into tissue. High-intensity focused ultrasound, as opposed to planar ultrasound, exceeds these diagnostic acoustic powers and deposits a much larger amount of energy (5000 and 100,000 times greater than those of diagnostic ultrasound) into the tissue. The increased wave intensity is focused, either geometrically or electronically (phased array), onto a single point. When the acoustic power at this focus point is sufficiently high, cavitation (microbubbles forming and interacting with the ultrasound field) will occur. Microbubbles produced in the field oscillate and grow (owing to factors including rectified diffusion), and eventually implode (inertial or transient cavitation). During the inertial cavitation, very high temperatures will occur inside the bubbles (65° to 85°C), destroying the tissue by coagulation necrosis. The internal cavitation is followed by a sudden and violent collapse of the microbubble that results in a shock wave, which causes additional mechanical damage to the tissue. Stable cavitation is the oscillation of existing microbubbles in the tissue and is not associated with a violent collapse and dispersion of energy. Microbubble oscillations can result in sheering forces and viscous-damping heating. Although stable cavitation is currently avoided during the procedure, there is some experimental evidence that stable cavitation may be able to enhance tissue ablation during HIFU and is being further investigated.
During HIFU, a reproducible but small volume of ablation is created for each pulse of energy. The geometry of each ablation volume is an ellipsoid, and the size of the ellipsoid is a function of crystal geometry. Through sequential movements of the applicator, the targeted volume is systematically ablated. Stacking these pulses of high intensity ultrasound will achieve this tissue destruction in as large or small of a volume as planned and desired ( Fig. 2 ). Tissues beyond the elementary ablation volume may heat slightly with more conduction perpendicular to the long axis of the elementary lesion than parallel to it. No ischemic infarction is produced by this technique. Cooling intervals are placed between each sonication so that the tissue anterior and, to a lesser extent, posterior to the focal spot cool between sonications, allowing further sonications to be placed closer to the first one without any damage to the intervening tissues. The passage of the HIFU beam through the tissues depends on the same physical factors as conventional diagnostic ultrasound. In other words, gas will reflect the HIFU beam and may cause areas of high heat build up at tissue-gas interfaces that must be avoided. Bone and calcifications will absorb HIFU energy very avidly, potentially shielding structures immediately behind them from heating but may also cause heat build up at the bone–soft tissue interface.
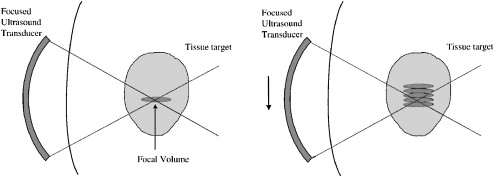
HIFU targeting and monitoring
Two methods are available to visualize, target, and monitor the tissue designated for destruction with HIFU: diagnostic ultrasound and magnetic resonance imaging (MRI). However, no single universally adhered method of using focused ultrasound (FUS) has yet emerged.
Diagnostic Ultrasound
The most common method to target and monitor HIFU therapy uses conventional diagnostic ultrasound transducers. Two separate crystals (therapeutic and diagnostic) can be packaged into one instrument ( Fig. 3 ). This allows for real-time monitoring of the target and a visual estimation of the acoustic coupling and any potential confounders of successful therapeutic HIFU delivery (air, bone, calcifications). This method allows a reasonable amount of flexibility in the targeting, but it does have several substantial drawbacks.
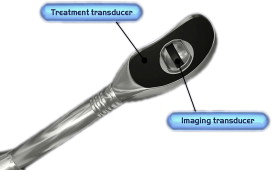
First, to fit both crystals into a single applicator, the applicator is larger and heavier than traditional diagnostic ultrasound probes. Size is a particular problem when designing applicators to be placed within natural orifices (eg, the esophagus, rectum, urethra, or uterus) rather than for transcutaneous application (eg, the liver, kidney, or pancreas). A smaller crystal has a smaller potential depth of the focal zone. As such, this can limit the ability to treat a greater depth of tissue penetration and limits treatment to organs proximal to the applicator (eg, smaller prostates). Diagnostic ultrasound also has low spatial resolution, limiting its accuracy for targeting (in comparison with MR imaging). In addition, when there is bowel and air in the vicinity, it may be hard to visualize the details of adjacent structures around these gas-containing portions, as well as behind them, so that targeting may be limited. Finally, thermal mapping is difficult with ultrasound. Under ultrasound observation, the tissue temperatures are estimated through observation of changes in the tissue echogenicity and induction of microbubbles and cavitation. Although there are a variety of techniques becoming gradually available that may improve the thermal resolution of ultrasound, these techniques remain quite cumbersome. Despite these relative drawbacks, HIFU with ultrasound guidance has been used to treat many patients, particularly in the Far East, in a variety of applications and shows substantial promise in these areas.
Magnetic Resonance Imaging
Developing HIFU machinery that works in the extremely hostile environment of an MR scanner is a substantial technical achievement. No ferromagnetic material may be used and radiofrequency leakage from electrical components will cause substantial MR image degradation. Simple motion of the transducer has to be accomplished by MR-compatible means, such as the use of piezoelectric motors rather than conventional electric motors. Substantial filtering of radiofrequency leakage together with shielding of electric connections must be used to limit interference with imaging.
Imaging with MR offers excellent visualization of the full extent of tumor within its tissue structure and depicts the boundary of soft tissues very clearly. It is precisely this ability that is so useful in the targeting of HIFU and where diagnostic ultrasound guidance alone is most problematic. Additionally, MR allows real-time thermal resolution with high spatial resolution ( Fig. 4 ). Therefore, thermal maps are rapidly and easily acquired with MR and are accurate to ± 2°C using conventional phase shift imaging. Several MRI parameters are temperature sensitive and allow detection of small temperature elevations before any induced irreversible tissue damage. Thus, the focus can be located at relatively low powers, and the accuracy of targeting can be verified. In addition, by using temperature-sensitive MRI sequences, focal temperature elevations and effective thermal doses may be estimated. An added advantage is that MRI is often used for preoperative and postoperative assessment of the targeted area so that consistent imaging platforms can be used to monitor therapeutic results. However, MRI is expensive and bulky. The treatment time is also prolonged, because despite its imaging advantages, the acquisition of images is much more time consuming than ultrasound.
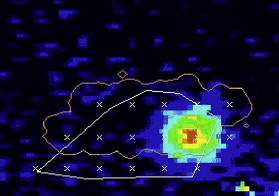
HIFU targeting and monitoring
Two methods are available to visualize, target, and monitor the tissue designated for destruction with HIFU: diagnostic ultrasound and magnetic resonance imaging (MRI). However, no single universally adhered method of using focused ultrasound (FUS) has yet emerged.
Diagnostic Ultrasound
The most common method to target and monitor HIFU therapy uses conventional diagnostic ultrasound transducers. Two separate crystals (therapeutic and diagnostic) can be packaged into one instrument ( Fig. 3 ). This allows for real-time monitoring of the target and a visual estimation of the acoustic coupling and any potential confounders of successful therapeutic HIFU delivery (air, bone, calcifications). This method allows a reasonable amount of flexibility in the targeting, but it does have several substantial drawbacks.
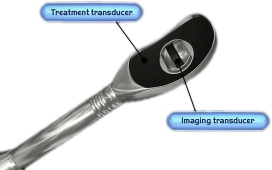
First, to fit both crystals into a single applicator, the applicator is larger and heavier than traditional diagnostic ultrasound probes. Size is a particular problem when designing applicators to be placed within natural orifices (eg, the esophagus, rectum, urethra, or uterus) rather than for transcutaneous application (eg, the liver, kidney, or pancreas). A smaller crystal has a smaller potential depth of the focal zone. As such, this can limit the ability to treat a greater depth of tissue penetration and limits treatment to organs proximal to the applicator (eg, smaller prostates). Diagnostic ultrasound also has low spatial resolution, limiting its accuracy for targeting (in comparison with MR imaging). In addition, when there is bowel and air in the vicinity, it may be hard to visualize the details of adjacent structures around these gas-containing portions, as well as behind them, so that targeting may be limited. Finally, thermal mapping is difficult with ultrasound. Under ultrasound observation, the tissue temperatures are estimated through observation of changes in the tissue echogenicity and induction of microbubbles and cavitation. Although there are a variety of techniques becoming gradually available that may improve the thermal resolution of ultrasound, these techniques remain quite cumbersome. Despite these relative drawbacks, HIFU with ultrasound guidance has been used to treat many patients, particularly in the Far East, in a variety of applications and shows substantial promise in these areas.
Magnetic Resonance Imaging
Developing HIFU machinery that works in the extremely hostile environment of an MR scanner is a substantial technical achievement. No ferromagnetic material may be used and radiofrequency leakage from electrical components will cause substantial MR image degradation. Simple motion of the transducer has to be accomplished by MR-compatible means, such as the use of piezoelectric motors rather than conventional electric motors. Substantial filtering of radiofrequency leakage together with shielding of electric connections must be used to limit interference with imaging.
Imaging with MR offers excellent visualization of the full extent of tumor within its tissue structure and depicts the boundary of soft tissues very clearly. It is precisely this ability that is so useful in the targeting of HIFU and where diagnostic ultrasound guidance alone is most problematic. Additionally, MR allows real-time thermal resolution with high spatial resolution ( Fig. 4 ). Therefore, thermal maps are rapidly and easily acquired with MR and are accurate to ± 2°C using conventional phase shift imaging. Several MRI parameters are temperature sensitive and allow detection of small temperature elevations before any induced irreversible tissue damage. Thus, the focus can be located at relatively low powers, and the accuracy of targeting can be verified. In addition, by using temperature-sensitive MRI sequences, focal temperature elevations and effective thermal doses may be estimated. An added advantage is that MRI is often used for preoperative and postoperative assessment of the targeted area so that consistent imaging platforms can be used to monitor therapeutic results. However, MRI is expensive and bulky. The treatment time is also prolonged, because despite its imaging advantages, the acquisition of images is much more time consuming than ultrasound.
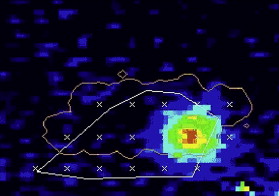
The HIFU equipment
Magnetic Resonance–Guided Focused Ultrasound
Gadolinium-enhanced T1-weighted images clearly show the extent of necrosis following an HIFU treatment. MRI has also been used to guide HIFU treatment and to monitor temperature changes during HIFU (see Fig. 4 ).
Currently, only one commercially available MR-compatible HIFU machine is available (ExAblate 2000, Insigtec, Haifa, Israel), although several other prototypes are currently being tested (Phillips Healthcare, Amsterdam, The Netherlands).
The ExAblate 2000 system consists of 4 components, which all work in synergy. These are the MR scanner, the HIFU table containing the transducer and water bath, the electronic components driving the system, and the HIFU workstation. The latter links to the MR workstation and allows user interaction. To date, this system works only on the General Electric (Fairfield, CT, USA) MRI scanner platform. The HIFU table is identical to the conventional MR table, with the addition of a built-in transducer and water bath, and electronic connections. The current machinery contains a 211-phased array element within a water bath. The transducer may be moved by its robotic system to any position in the water bath, and the depth of focus can be varied electronically to allow great flexibility of positioning. The transducer frequency is variable, ranging from 0.9 to 1.4 MHz.
Recently, the combination of planar ultrasound transducers under rotational control with active MR temperature feedback that can produce conformal spatial patterns of thermal damage within the prostate gland has been described. To achieve this, a device containing the piezoelectric treatment crystal is inserted into the urethra and delivers high-intensity ultrasound energy to adjacent prostate tissue. MR imaging is used during treatment to measure the spatial temperature distribution in the prostate and surrounding tissues noninvasively. This unique capability to measure the temperature distribution in the prostate gland continuously during treatment enables adaptive therapy delivery that can compensate for changes in blood flow or ultrasound properties of the prostate gland that are known to occur during heating. In addition, quantitative knowledge of the amount of heating in surrounding tissues can be used to protect critical structures (rhabdosphincter, erectile nerves, rectum) from thermal damage. A prototype system for MRI-guided transurethral ultrasound therapy within a conventional closed-bore MR environment is now being developed by Profound Medical Inc (Toronto, Canada).
Ultrasound-Guided HIFU for Prostate Cancer Treatment
Current transducers for transrectal prostate applications use single-focus transducers that are moved mechanically. There are 2 HIFU technologies currently used for the treatment of prostate cancer, although neither one is yet approved by the Food and Drug Administration (FDA). The first developed was the Ablatherm (Edap-Technomed, Lyon, France). Subsequently, Focus Surgery Inc (Indianapolis, IN, USA) developed a system called the Sonablate. The Ablatherm consists of a specific bed containing the ablation technology upon which the patient lies in a lateral position. For the Sonablate system, the procedure is conducted in a lithotomy position.
The Ablatherm includes 3 predefined treatment protocols with specifically designed energy parameters depending on the clinical use (Primary therapy, HIFU retreatment, and failure after prior radiation) ( Fig. 5 A, B). The Sonablate uses a single treatment protocol in which the operator manually adapts the power. This “visually directed” HIFU treatment is based on gray-scale ultrasonographic changes observed during treatment that allow the operator to change the power level for each HIFU pulse. The underlying science and technology of both systems is identical but differences from different schools of thought with regard to how to best design the optimal HIFU treatment system have led to very different machines. Specifically, the differences arise in how the manufacturers choose optimal ultrasonic frequencies and powers, as well as differences on the optimal method for proceeding through the treatment plan. These differences have in turn led to differences in image quality and treatment-induced cavitation. Increasing the frequency increases the incidence of cavitation, increases image resolution near the ultrasound crystal, but reduces ultrasound penetration. The fundamental physical constraint in the design of a transducer is the fact that it must be inserted into the rectum during treatment. The physical size and shape of the ultrasound transducer determines where the energy can be focused.
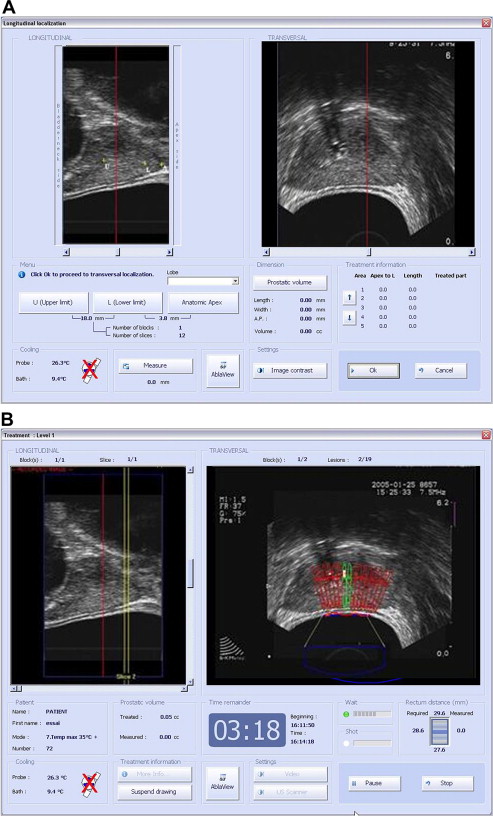
The Ablatherm uses separate crystals for imaging (7.5 MHz) and treatment (3.0 MHz). Thus, the dependence of image resolution is removed from the equation when ablation is occurring. Imaging probes for standard prostate applications tend to range from 5.0 MHz to 7.5 MHz, with higher frequency probes creating higher quality images. The real-time 7.5-MHz probe used by the Ablatherm creates a very high-quality image throughout the prostate and a 3.0-MHz treatment probe was determined to be the best for treatment. Thus, optimal values are used for both imaging and treatment.
The Sonablate 500 uses a single crystal for both imaging and treatment: a concave rectangular element cut from a spherical crystal surface that has a central 10-mm diameter segment used for imaging. There is no need to change probes between imaging and treatment; however, this constrains the probe to be of a single frequency, as probe frequency is characteristic of the crystal. An operating frequency of 4 MHz was determined to provide an acceptable compromise between image quality and effective treatment. The 4-MHz resolution probe allows for imaging of the anterior part of the prostate but has decreased resolution and image quality in the posterior margin of the gland and the rectal wall in comparison with higher frequency ultrasound probes.
The Ablatherm uses a single treatment probe that has a focal point 45 mm from the crystal. The 3-MHz probe creates an ablation volume size that is adjustable from 24.0 (anterior to posterior) × 1.7 × 1.7 mm (total volume = 36 mm ) down to 19.0 × 1.7 × 1.7 mm (total volume = 29 mm ). The intent is that a single pulse will result in an ablation that extends the entire anterior to posterior height of the prostate. This strategy has the advantage that only one focus is needed to treat the entire height of the gland, but given that prostates are not uniform in height (the base is taller than the apex), it can result in the ablation of some tissue beyond the anterior margin of the prostate.
The Sonablate probe is composed of 2 different crystals with different focal lengths. This is accomplished by having 2 crystals placed back to back within the probe, one with a 3-cm focal length and the other with a 4-cm focal length. The discrete ablation volume of both these crystals is 10 (anterior to posterior) × 2 × 2 mm (total volume = 21 mm ). Because of the decreased height of the ablation zone, complete anterior to posterior ablation is not usually possible with a single pulse of energy. The advantage to this strategy is that the reduced ablation volume provides better conformation to the anterior margin of the prostate. The disadvantage is time, because 2 to 3 planes of treatment from anterior to posterior throughout the whole gland are required, as most every prostate has an anterior posterior height in excess of 10 mm.
The Ablatherm device provides many safety features, including a safety ring that stabilizes the rectum wall, a permanent control of the distance between the therapy transducer and the rectal wall, and a patient motion detector. The Sonablate requires a permanent presence of the physician during the procedure to control the adequacy between what was planned and what is done.
Ultrasound-Guided Transcutaneous HIFU
Currently, only Haifu Technology Company (Chongqing, China) produces an ultrasound guided/monitored HIFU system for the treatment of a variety of benign and malignant solid organ tumors (uterine fibroid, benign breast neoplasm, benign tumors of soft tissues, malignant bone tumor, liver cancer, sarcoma of soft tissue, renal cancer, breast cancer, bladder caner, pancreatic cancer, tumor metastasis). This system consists of 3 selectable therapeutic transducers and a real-time imaging transducer. The transducers are mounted in a water reservoir with the beam axis directed upward, and the patients positioned above the transducers in a prone or decubitus position. Although certified by the European Union for the treatment of benign and malignant soft tissue tumors, this technology is not yet available in North America even through clinical trials, and is currently available in 3 European and 4 Asian centers. Theoretically, this system would be less costly and time consuming than MR-guided devices.
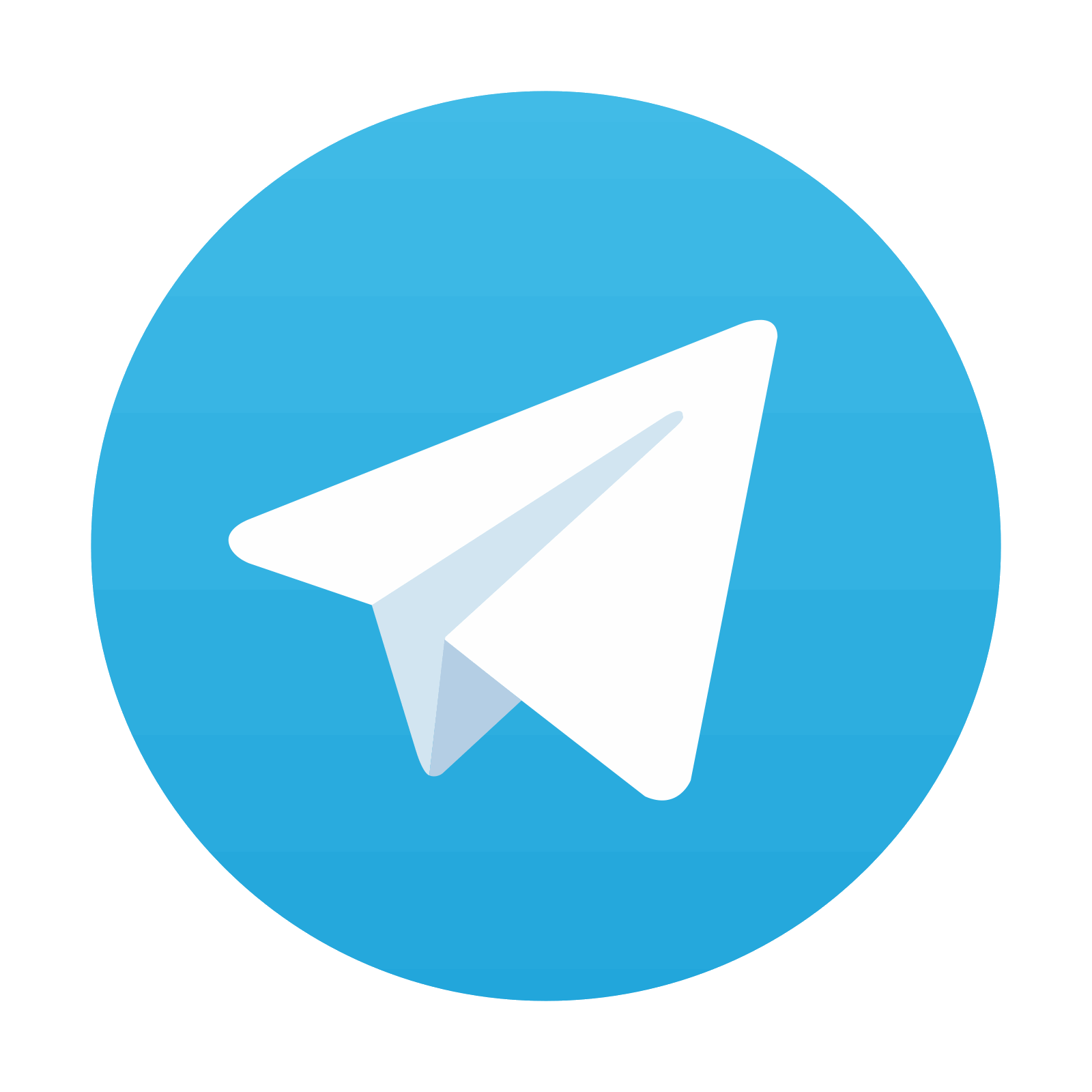
Stay updated, free articles. Join our Telegram channel
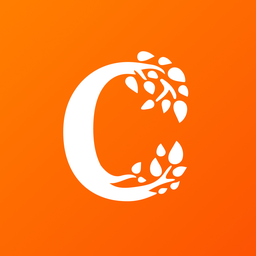
Full access? Get Clinical Tree
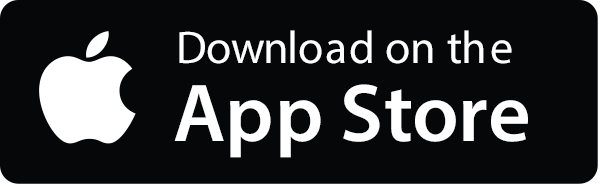
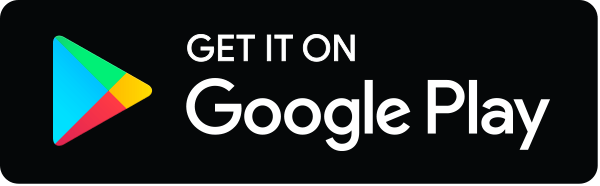