Different pathways lead from the simple point mutation in hemoglobin to the membrane changes that characterize the altered interaction of the sickle red blood cell with its environment, including endothelial cells, white blood cells, and platelets. Polymerization and oxidation-induced damage to both lipid and protein components of the red cell membrane, as well as the generation of bioreactive membrane material (microparticles), has a profound effect on all tissues and organs, and defines the vasculopathy of the patient with sickle cell disease.
Key points
- •
Although the pathophysiology of sickle cell disease may be uniquely related to the polymerization of sickle hemoglobin under low oxygen conditions, it has become apparent that many factors are involved in the vasculopathy that characterizes this disorder affecting millions of individuals worldwide.
- •
The altered red blood cell (RBC) membrane plays an important role in the dysfunctional interactions of the sickle RBC with other blood cells and vascular endothelium, and leads to premature recognition and removal, an imbalance in hemostasis, vaso-occlusive events, and intravascular hemolysis, and may be involved in acute chest syndrome.
- •
The complex, well-orchestrated RBC membrane phospholipid organization is apparently lost in subpopulations of RBC during erythropoiesis as well as in the circulation. Increased oxidant stress may play an important role in the inability of the RBC to maintain composition and asymmetry in phospholipid molecular species, but the mechanisms that lead to phosphatidylserine (PS) exposure are poorly understood.
- •
This lack of knowledge is in part due to the incomplete characterization of the proteins involved in the maintenance of phospholipid asymmetry in the RBC, as well as the complexity of studying a complete plasma membrane in which several protein entities act in synchronization with each other and are governed by protein-protein and protein-lipid interactions.
- •
The purification and/or expression of the proteins thought to be involved in membrane organization in well-defined lipid bilayers, their 3-dimensional structural modeling, and detailed functional characterization may lead to a better understanding of their individual functions and their interaction with other entities in the bilayer.
- •
This knowledge will also lead to a better understanding of how the function of these proteins is impaired or altered in leading to PS exposure in hemoglobinopathies, and to a definition of the molecular underpinnings of this complex pathophysiology.
Introduction
It has been 100 years since Herrick published the first medical case report of the anemia describing abnormal shapes of red blood cells (RBCs) and gave sickle cell anemia its name. In 1949, Pauling and Itano defined the underlying molecular reason by identifying hemoglobin S (HbS), and defined sickle cell anemia as the first “molecular disease.” The last 60 years have resulted in an increasingly coherent detailed molecular-level description of the pathophysiology of sickle cell disease (SCD). While great progress has been made in describing the basic disease process that accounts for hemolytic anemia and the obstructive events underlying vaso-occlusive events (VOE), many questions remain. The simple mutation in the β6 location of globin has a profound effect on all tissues and organs in the SCD patient, and because the vasculopathy affects a large variety of physiologic mechanisms, the varied genetic background of individual patients makes prediction of the clinical severity highly complex. However, it assuredly starts with the mutated hemoglobin (Hb) and the changes in the membrane that result from it. Hb is a complex molecule that undergoes conformational changes in response to oxygen, and is affected by environmental changes, allosteric effectors, and mutations. The polymerization of deoxy HbS, which forms long fibers inside the RBC, leads to the typical distorted sickle RBC (SRBC) morphology that was noted by Herrick. Together with changes in cytosol, which result from the relatively unstable pro-oxidant character of HbS, the morphology and ability of the SRBC to deform are affected. Both the mechanical stress on the RBC membrane and oxidation-induced damage affect both lipid and protein components of the RBC membrane and alter the interaction of the SRBC with its environment, including endothelial cells, white blood cells, and platelets, and leads to the loss of bioactive membrane material (particles). A loss of normal ion permeability of the SRBC membrane leads to an altered hydration status of the cell. In turn this affects polymerization, and an altered cytosolic calcium status affects a variety of processes, which will affect the plasma membrane and lead to apoptosis during erythropoiesis, in addition to hemolysis and early removal of the adult SRBC. While different pathways may lead from the simple point mutation in hemoglobin to the membrane alterations, the changes in the SRBC membrane and the downstream effects on its environment result in the vasculopathy that characterizes the disease.
Introduction
It has been 100 years since Herrick published the first medical case report of the anemia describing abnormal shapes of red blood cells (RBCs) and gave sickle cell anemia its name. In 1949, Pauling and Itano defined the underlying molecular reason by identifying hemoglobin S (HbS), and defined sickle cell anemia as the first “molecular disease.” The last 60 years have resulted in an increasingly coherent detailed molecular-level description of the pathophysiology of sickle cell disease (SCD). While great progress has been made in describing the basic disease process that accounts for hemolytic anemia and the obstructive events underlying vaso-occlusive events (VOE), many questions remain. The simple mutation in the β6 location of globin has a profound effect on all tissues and organs in the SCD patient, and because the vasculopathy affects a large variety of physiologic mechanisms, the varied genetic background of individual patients makes prediction of the clinical severity highly complex. However, it assuredly starts with the mutated hemoglobin (Hb) and the changes in the membrane that result from it. Hb is a complex molecule that undergoes conformational changes in response to oxygen, and is affected by environmental changes, allosteric effectors, and mutations. The polymerization of deoxy HbS, which forms long fibers inside the RBC, leads to the typical distorted sickle RBC (SRBC) morphology that was noted by Herrick. Together with changes in cytosol, which result from the relatively unstable pro-oxidant character of HbS, the morphology and ability of the SRBC to deform are affected. Both the mechanical stress on the RBC membrane and oxidation-induced damage affect both lipid and protein components of the RBC membrane and alter the interaction of the SRBC with its environment, including endothelial cells, white blood cells, and platelets, and leads to the loss of bioactive membrane material (particles). A loss of normal ion permeability of the SRBC membrane leads to an altered hydration status of the cell. In turn this affects polymerization, and an altered cytosolic calcium status affects a variety of processes, which will affect the plasma membrane and lead to apoptosis during erythropoiesis, in addition to hemolysis and early removal of the adult SRBC. While different pathways may lead from the simple point mutation in hemoglobin to the membrane alterations, the changes in the SRBC membrane and the downstream effects on its environment result in the vasculopathy that characterizes the disease.
Polymerization
As the predominant cell type, the RBC largely determines the rheologic and hemodynamic behavior of blood. The intricate mechanisms that govern the interaction of the RBC membrane skeleton with membrane proteins and the lipid bilayer provide the ability of the RBC to deform under shear stress in the circulation and regain its typical shape as a biconcave disk. In SCD, mechanically fragile, poorly deformable RBCs contribute to impaired blood flow and other pathophysiologic aspects of the disease. Formation of hemoglobin polymers in the SRBC negatively affect the RBC’s ability to maintain its normal morphology, and it has long been considered that the radical shape change of the SRBC under low oxygen leads to the inability of the SRBC to properly deform and pass though the microvasculature, leading to VOE. In addition to the change in shape, HbS polymer formation will cause mechanical stress on the RBC membrane, resulting in membrane changes as well as loss of membrane material, evidenced by the increased circulating RBC-derived microparticles (MPs). The kinetics of HbS polymerization are affected by the presence of normal Hb (HbA) or fetal Hb (HbF). Although sickle cell trait individuals (HbAS) can experience VOE, this is a rare event and seems related to extreme dehydration or low oxygen tension as experienced at higher altitudes. The replacement of 50% of HbS by HbA slows polymerization by approximately 100-fold. Increased levels of gammaglobulin ameliorate the severity of SCD because HbF can effectively replace HbS and lower the rate of polymerization, providing a treatment protocol by reversing the Hb switch that occurs before birth. The success of hydroxyurea (HU) treatment is mainly based on increasing HbF levels, but the understanding of the molecular mechanism of globin switching is still unclear. In addition to HbF modulation, HU has been implicated in affecting several different genes, the SRBC proteome, and other mechanisms including adhesion, phosphatidylserine (PS) exposure, and fatty acid metabolism. Given the central role of HbS polymerization, several approaches aimed at affecting gammaglobulin production have been explored, including the use of decitabine, butyrate, lenalidomide, and pomalidomide (see later discussion). Increased understanding of the factors that regulate gammaglobulin expression provides potential novel approaches, such as the recently identified chromatin factor Friend of Prmt1 (FOP), a critical modulator of gammaglobulin gene expression. Of importance, the complex genetic background of the sickle cell patient that seems to affect the clinical course of the disease has also led to the identification of specific single-nucleotide polymorphisms (SNPs) related to the expression of HbF. The repressor BCL11A, in particular, seems important in defining the response to hydroxyurea treatment. Because the state of RBC hydration links directly to polymerization, drugs have been tested that decrease intracellular HbS concentration by increasing total cell volume by modulating membrane ion-exchange channels, such as the Gardos Channels and the KCL Cotransporter. These compounds include magnesium pidolate, imidazole antimycotics, arginine, and Senicapoc. In addition to the types of Hb that make up the Hb mixture in the SRBC, the rate of polymerization is strongly affected by the interface between the 2 α/β dimers, exposed in the deoxy state. Factors that modulate this interaction will affect polymerization; the excessive amount of heme released in SRBCs is one of these factors. Free heme at micromolar concentrations induces strong attraction between the hemoglobin molecules, and drastically increases rates of nucleation and polymer fiber growth. In addition to the rate of polymerization, it is also important that modulation of the kinetics of depolymerization is essential to achieve full dissolution of polymers in the lungs or resolution of VOE. Conditions whereby residual HbS polymers exist facilitate repolymerization and thus may be related to abnormality. Because deoxygenation drives the polymerization of HbS, mutations that affect the oxygen-binding equilibrium will likely affect the sickling process. A small number of these mutations occur in 3 predicted druggable binding pockets that might be exploited to directly inhibit polymerization. Compounds that modulate oxygen affinity are tested for their efficacy to increase oxygen affinity and decrease HbS polymerization (see later discussion). Although some studies have been promising in a transgenic sickle animal model, there is a paucity of data from human studies. A promising antisickling agent is yet to be established. In addition, mechanisms that are normally physiologically active in modulating oxygen affinity are exploited. Such mechanisms include hypoxia-mediated elevated adenosine signaling (see later discussion). In addition to polymerization under low oxygen, it has to be taken into account that the oxygenation state of the RBC affects many aspects of RBC metabolism, owing to the interaction of (deoxygenated) Hb and membrane components. Band 3, an integral membrane protein linked to the spectrin/actin cytoskeleton, preferentially binds to deoxygenated Hb at its N-terminus, and affects the association between band 3 and the underlying RBC cytoskeleton. This process affects the generation of adenosine triphosphate (ATP), hydrogenated nicotine adenine dinucleotide (NADH), 2,3-diphosphoglycerate (DPG), and NADH phosphate (NADPH) as the result of competition between deoxyhemoglobin and key Embden-Meyerhof pathway enzymes for binding to the cytoplasmic domain of band 3. In oxygenated RBCs, NADPH generation maximizes glutathione-based antioxidant systems through the hexose monophosphate pathway. A constrained hexose monophosphate pathway flux, caused by| an abnormal oxygen-dependent association of sickle hemoglobin with the RBC membrane, interferes with the regulation of NADPH. This reduced resilience to NADPH, and glutathione recycling and oxidative stress, is manifested by membrane protein oxidation and membrane fragility, suggesting that hypoxia may influence the SCD phenotype independent of polymerization.
Effects of HbS on membranes
The presence of HbS results in many changes in the SRBC cytosol and its membrane, including an altered proteomic or metabolomics profile, redox status, altered ion transport, changed adhesive properties (see later discussion), and loss of membrane lipid asymmetry, resulting in PS exposure. Metabolic signatures of specialized circulating hematopoietic cells using liquid chromatography–mass spectrometry based metabolite profiling reveal that RBC and SRBC metabolomes display major differences in glycolysis, glutathione, ascorbate metabolism, and metabolites associated with membrane turnover. In addition, the amounts of metabolites derived from the urea cycle and nitric oxide metabolism that partly take place within RBCs seem different. In part this is related to factors that maintain the redox status and oxygen affinity.
A complicating factor is that SRBCs form a highly heterogeneous population, and membranes are altered differently in subpopulations of RBCs, which makes it extremely difficult to link clinical observations or membrane changes to the average SRBC. The SRBC population includes a wide range of young-deformable to rigid-irreversible SRBCs, as well as cells with different states of hydration. The RBC membrane is endowed with a large variety of ion channels. Although the physiologic role of several of these channels remains unclear and inactive in the resting cell, when activated experimentally these ion channels can lead to a very high single-cell conductance and potentially induce disorders, with the major risks of rapid dehydration and dissipation of gradients. The presence of circulating dense RBCs is important in SCD-related clinical manifestations, but the large range of densities in SRBCs indicates that the membrane alterations that lead to permeability changes of the SRBC vary significantly from cell to cell. The SRBC population exhibits heterogeneous adhesive behavior attributable to the diverse cell morphologies and membrane properties. More adhesive SRBCs interact with the vascular endothelium and trap irreversible sickle cells (ISCs); similarly, adherent leukocytes may also trap ISCs resulting in vaso-occlusion in small and even larger vessels. Although the presence of only a small percentage of PS-exposing cells may sound less important, the prothrombotic PS-exposing surface that these SRBCs provide is very significant in comparison with, for example, the total number of platelets in the circulation.
Furthermore, it is difficult to separate the effects of polymerization from the other changes induced by HbS, including oxidant stress. Oxidant stress will alter the RBC membrane such that additional mechanical stress will result in much larger changes. The altered cytosolic composition of the SRBC leads to several downstream effects. For example, the loss of proper calcium homeostasis in SRBCs will affect several pathways. Ca 2+ is a universal signaling molecule involved in regulating cell cycle and fate, cell metabolism and structural integrity, and cell motility and volume. Like other cells, erythroid cells, during development and in the circulation, rely on Ca 2+ -dependent signaling. Intracellular Ca 2+ levels in the circulating human RBCs take part not only in controlling biophysical properties such as membrane composition, volume, and rheologic properties, but also physiologic parameters such as metabolic activity, redox state, PS exposure, and cell clearance.
Oxidative stress
A common denominator in the alterations in the SRBC and the damage to the RBC membrane is the well-recognized increased HbS auto-oxidation and Fenton chemistry reactions catalyzed by denatured heme moieties bound to the RBC membrane, further enhanced by NADPH oxidase, which is regulated by protein kinase C, Rac guanosine triphosphatase, and intracellular Ca 2+ signaling within the sickle RBC (see later discussion). The extremely high level of oxidant stress in SRBC or thalassemic RBC leads to higher levels of damage and premature aging of these cells. Footprints of increased oxidant damage such as methemoglobin (MetHb), lipid peroxidation products, or altered protein thiol status, are found in both thalassemic RBCs and SRBCs. The main function of the RBC, oxygen transport, requires a special skill set to deal with the oxidant stress that results from this activity. The continuous process of Hb oxygenation and deoxygenation is accompanied by transient and reversible changes in heme iron redox state from the ferrous (Fe 2+ ) to the ferric (Fe 3+ ) state, with small amounts of superoxide being produced in the process. A complex system of antioxidants and enzymes aims to protect the RBC from oxidant-induced damage. These agents include enzymes such as superoxide dismutase, catalase, thiol-active reagents (glutathione, peroxyredoxin, ergothionine), lipid antioxidants (vitamin E), and enzyme systems that can regenerate oxidized compounds using RBC metabolism, as well as systems that can repair lipid and protein damage. The latter are important, as the RBC is unable to generate new protein and lipid, and despite the antioxidant systems in place, damage to lipids and proteins does occur. These oxidant-induced alterations in structure and function of the RBC need to be repaired, and the inability to do so will lead to the demise of the cell and is in part responsible for the limited life span of the RBC. Superoxide generated by hemoglobin is transformed to hydrogen peroxide (H 2 O 2 ) by superoxide dismutase and subsequently reduced by catalase to water. Superoxide can cause the formation of ferric methemoglobin (metHb), which in turn is reduced back to its oxygen-binding ferrous state by metHb reductase. One percent to 2% of the hemoglobin exists as metHb in normal cells, which in turn is the starting point of the enzymatic reaction that leads to the formation of hemoglobin peroxidase. In the presence of H 2 O 2 , metHb act as peroxidase and catalyzes the 2-electron reduction of H 2 O 2 to water from oxidizing other biological cosubstrates (DNA, lipids, and proteins) in the process. During this process, H 2 O 2 (or lipid hydroperoxide) oxidizes ferric heme to perferryl heme and forms a protein-centered radical. The catalytic redox cycling between the perferryl and ferric heme makes a potent peroxide-fueled radical generator. Both perferryl and the protein-centered radicals are highly reactive, like hydroxyl radicals, and are capable of oxidizing biological macromolecules. Several thiol-based antioxidant systems are well described in the literature while others are less well studied. The primary defense against heme peroxidase activity is the efficient removal of H 2 O 2 . In addition to the catalase activity, peroxiredoxin II (Prx II) is a noncatalytic scavenger of H 2 O 2 , which at 250 μM is the second or third most abundant protein expressed in RBCs. Prx II is the principal protein responsible for detoxifying endogenously generated H 2 O 2 , but during the course of peroxidation the catalytic cysteine residue of Prx II becomes overoxidized and must be regenerated in a thioredoxin-dependent manner. The rate of Prx II regeneration is slow and limits its efficiency in scavenging peroxides at higher concentrations. Consistent with the importance in RBC biology, Prx II knockout mice are anemic and exhibit an elevated rate of RBC turnover, and experience higher rate of oxidative damage. Ergothioneine (ergo), a thiol antioxidant, is predominantly synthesized in fungal organisms and is the second most abundant RBC thiol compound. However, the reasons for this abundance in hematopoietic tissues has not been investigated. Interestingly HbS, which increases Hb pro-oxidant activity, selectively depletes ergo over glutathione, suggesting that ergo has a specialized glutathione-independent function in protecting RBCs against pro-oxidative hemoglobin. Humans lack the capacity to synthesize ergo, but the presence of a highly efficient ergo transporter (Organic Cation Transporter Na Dependent 1; OCTN1) ensures its accumulation and retention across different tissues and cell types. Because ergo is derived strictly from diet, it may potentially be an essential (or conditionally essential) dietary micronutrient. At physiologic pH, ergo exists primarily as a thione and acts as a weak antioxidant. Therefore, it is unlikely that its primary role would be its radical scavenging ability. Its weak redox activity also minimizes its pro-oxidant interactions with transition metals and protein-SH moieties. Therefore, cells can accumulate ergo in large quantities without toxicity. It seems logical to assume that ergo is a specialized antioxidant for protecting maturing hematopoietic cells and mature RBCs. A major part of its pro-oxidative potential involves its ability to act as a pseudoperoxidase in the presence of peroxides. High expression of the ergo transporter in hematopoietic precursor cells relative to other major tissues, and increased expression in maturing erythroid cells that produce high levels of hemoglobin, corroborate the hypothesis of a critical antioxidant role of ergo during hematopoiesis and in determining the health of mature RBCs. The ability to deal with oxidant stress is compromised and challenged in SCD, resulting in an unbalanced redox state, an altered thiol redox metabolism, and protein and lipid damage. In addition to the altered redox status inside the RBC, the increased levels of hemolysis observed in SCD, as well as iron overload resulting from anemia and transfusion therapy, adds to the oxidant-induced tissue damage. Plasma proteins such as hemopexin and haptoglobin bind to cell-free heme and hemoglobin, and minimize their toxicity. In addition, the prompt induction of the heme-catabolizing enzyme, heme oxygenase-1, during oxidative stress conditions allows cells to attenuate heme stress by degrading excess heme to bilirubin and carbon monoxide gas. An antioxidant system specifically designed for inhibiting heme peroxidase activity has not been clearly identified. This factor seems particularly important, as hemoglobin peroxidase is a potent catalyst for damage following hemolysis observed in SCD. In addition to SCD, nonspecific tissue damage from heme/hemoprotein peroxidase activity has been detected in conditions such as myocardial infarcts and cerebral hemorrhage. In all of these cases, myoglobin or hemoglobin released from dying tissues or the RBCs act as peroxidase and initiate nonspecific oxidation of proteins, lipids, and DNA. This mechanism is further illustrated by the presence of myoglobin/hemoglobin cross-linked products in biological fluids (plasma and cerebral spinal fluids) following rhabdomyolysis (muscle damage) or subarachnoid hemorrhage. These cross-linked products can only be created when protein-centered radicals are formed during myoglobin/hemoglobin peroxidase reactions. In Alzheimer disease, high levels of β-amyloid in the brain can bind tightly to heme and act as a peroxidase, causing damage to surrounding neurons in the presence of excess hydrogen peroxide, and hemoglobin peroxidase activity has been shown to exacerbate atherogenesis by promoting plaque lipid oxidation and endothelial cell damage. Although these conditions may not seem to be related to the sickle cell mutation, it has to be considered that the multiorgan damage typical of SCD has components that are closely related, and it needs to be considered that treatment regimens used for these disorders may also be applicable to SCD.
Microparticles
MPs are submicron membrane vesicles or “membrane dust,” shed by compromised cells including RBCs. MPs have been described as biomarkers in various vascular diseases, including SCD, and have been associated with an increased risk of thrombosis. Although decline of HbF coincided with an increase in circulating MPs, treatment with hydroxyurea showed lower concentrations of total MPs shed by platelets and RBCs. The increased level of MPs in SCD is linked to intravascular hemolysis, as shown by the correlation between levels of MPs and plasma Hb. In addition, subpopulations of RBCs expressing PS were associated with MPs, and HU treatment decreased these parameters in comparison with untreated controls. Band 3, an integral membrane protein linked to the spectrin/actin cytoskeleton, preferentially binds to deoxygenated Hb at its N-terminus, and affects the association between band 3 and the underlying RBC cytoskeleton. The displacement of ankyrin from band 3 leads to release of the spectrin/actin cytoskeleton from the membrane, and is able to promote unwanted membrane vesiculation and the formation of RBC-derived MPs. The cytoplasmic domain of band 3 serves as a center of RBC membrane organization and constitutes the major substrate of RBC tyrosine kinases. Tyrosine phosphorylation of band 3 is induced by several physiologic stimuli, including malaria parasite invasion, cell shrinkage, normal cell aging, and oxidant stress such as occurs in SCD. Oxidative insult, and band 3 oligomers that result, contribute to the adhesive nature of SRBCs with regard to endothelial cells. Because release of band 3 from its ankyrin and adducin linkages to the cytoskeleton can facilitate changes in multiple membrane properties, tyrosine phosphorylation of band 3 is argued to enable adaptive changes in RBC biology that permit the cell to respond to stress.
In addition to stress from the inside of the SRBC, external factors also have been implicated in the formation of this membrane dust. Thrombospondin 1 (TSP1) triggered rapid RBC conversion into echinocytes in vitro, followed by MP shedding. In a sickle mouse model, TSP1 led to the formation of PS, stimulating MPs and initiating vaso-occlusions within minutes. These events could be countered by cloaking the PS-positive surfaces with annexin-V (PS on the membrane surface of RBCs is discussed in more detail later). The expression of PS on circulating MPs plays an important role in the etiology of the hypercoagulable state of SCD, as well as in the reduced life span of RBCs and adhesive interactions between RBCs and endothelium.
Membrane lipid
The lipid bilayer of the RBC is likely the best studied mammalian plasma bilayer, and has revealed a highly complex and dynamic system whereby a diverse compilation of molecules are continuously renewed, move rapidly in the plane and across the bilayer in a highly orchestrated fashion, providing the membrane proteins a proper environment and maintaining a proper barrier that separates cytosol from the cell’s environment. This system is altered in subpopulations of the SRBC population, leading to altered membrane surface characteristics and permeability. In turn this leads to the large variation in cellular hydration, interaction with other blood cells, and early demise of the SRBC. During the early stages of erythropoiesis, the more than 250 molecular species of glycerophospholipid and sphingomyelin (SM) that form the structural backbone of the lipid bilayer if the RBC are synthesized de novo. Although it can be expected that these molecular species are remodeled by deacylation and reacylation during the various stages of RBC development, virtually no data on these processes during erythropoiesis are available. In the last stage of RBC development, a portion of the membrane lipid bilayer during enucleation is lost and the reticulocyte is born. Further remodeling in the circulation results in additional loss of membrane material, and the adult RBC starts its life. The glycerophospholipid molecular species (phosphatidylcholine [PC], phosphatidylethanolamine [PE], and PS) together with SM and cholesterol, make up most of the plasma membrane lipids. Their rapid movement in the plane of the bilayer is highly organized and leads to areas that are enriched in specific phospholipid molecular species. These areas or microdomains provide a specific environment for membrane proteins to regulate the traffic of ions and signals across the bilayer. Across the bilayer lipids are highly asymmetrically distributed, with the aminophospholipids mainly (PE) or exclusively (PS) in the inner leaflet of the bilayer and the choline phospholipids (PC, SM) mainly in the outer leaflet. The mature mammalian RBC lacks internal organelles and is incapable of de novo lipid synthesis. Nevertheless, a very dynamic system maintains the overall composition and organization of the membrane during the life of the adult RBC. Lipid molecules are continuously renewed. Fatty acids are rapidly taken up from plasma and incorporated into phospholipids. Despite this rapid phospholipid turnover, and the continuous changes in the substrate pool (plasma fatty acids, determined by diet), the molecular species composition of RBC is remarkably constant. This stability indicates a highly selective system within which several components act in synchronization; it thus seems appropriate to hypothesize that the activities of these proteins are regulated by lipid-protein and/or protein-protein interactions in the membrane that “sense” the need for the generation of certain molecular species or the need for lipid (re-)distribution in the plane and across the bilayer. The mechanisms for these interactions are largely unknown, in part because many of the membrane proteins involved are poorly characterized. Similarly, whereas the exposure of PS on the surface of both adult RBC and RBC precursors is well recognized as an important contributor to SCD pathology, the mechanisms that lead to this exposure are still poorly defined.
Lipid turnover
The turnover of membrane fatty acyl groups and the redistribution of phospholipids across the bilayer is in part the result of the basic function of the RBC, the transport of oxygen, as already indicated. The high levels of reactive oxygen species (ROS) lead to damage of the polyunsaturated acyl groups in the different phospholipid classes despite the presence of a network of antioxidant systems. This aspect is particularly important when either antioxidants are compromised or, as is the case in SCD, when more ROS are generated. The polyunsaturated fatty acids in the phospholipids are prime targets for alterations in oxygen radicals. When oxygen reacts with the double bonds, it introduces a polar entity in the apolar chains. This breach in the normal membrane structure is recognized by phospholipases, which hydrolyze the ester bond and generate lysophospholipids, starting a repair process. Several proteins involved in the deacylation/reacylation mechanism (the so-called Lands pathway ) have been identified, including acyl–coenzyme A (CoA) synthetases, acyl-CoA binding proteins, and acyl-CoA transferases. The acyl-CoA synthetase ACSL6 in the membrane of the adult RBC activates fatty acids taken up from plasma by ligation of the fatty acid to CoA at the expense of ATP. Subsequently the acyl-CoA is transferred to lysophospholipid by members of the elusive lysophospholipid acyl-CoA acyltransferase (LPLAT) family. Different members of the LPLAT family have preferences for the different phospholipid headgroups, and different proteins may be responsible for the reacylation of lysophosphatidylcholine (LPC), lysophosphatidylethanolamine (LPE), and lysophosphatidylserine (LPS). The protein that reacylates LPC to PC in the RBC membrane was recently identified, and was shown to be sensitive to both calcium and oxidant stress. Oxidant damage is random in nature, and the phospholipid repair system should be able to replace the damaged phospholipid in the complex phospholipid pool with the same molecular species to maintain the bilayer structure. This process requires the ability to reacylate lysophospholipids using specific fatty acids from a substrate pool that changes depending on diet. The mechanisms that govern this well-regulated repair system are not known. Transbilayer movement is coupled with this reacylation process. The reacylation takes place in the inner monolayer, and proper redistribution of the molecular species is required, involving the proteins that transport phospholipids across the bilayer. Therefore, it is logical to assume that the redistribution of these phospholipid species across the bilayer, when they are generated at the inner leaflet, is also a specific process. A rapid translocation from inner to outer monolayer of newly synthesized PC but not PE is observed, suggesting a selective, protein-mediated, outward translocation of newly synthesized PC. The proteins involved in this repair process are themselves vulnerable to oxidant damage. Because the adult RBC is not able to replace damaged proteins, this may lead to the inability to properly repair damaged phospholipids, a dysfunction of the membrane, and demise of the cell.
Lipid asymmetry
The difference in phospholipid composition between the 2 leaflets of the mammalian RBC plasma membrane bilayer was first reported more than 3 decades ago This asymmetric transbilayer organization in the membrane of the RBC was originally regarded as a static distribution across the bilayer, generated during erythropoiesis and maintained during the life of the cell, in part by the interaction between PS and spectrin. Therefore, HbS polymerization and its mechanical stress on the membrane was thought to result in local loss of asymmetry. In 1984 it was shown that the asymmetric transbilayer distribution is the result of a dynamic and ATP-dependent transport process. The removal of virtually all of the PS from the surface of the plasma membrane seems typical for normal mammalian cells. The ATP-driven transbilayer movement in the RBC is specific for the PE and PS. The outside-to-inside movement, or flip of aminophospholipid in one direction and the flop of another molecule in the reverse to compensate, may involve a “floppase” or proteins such as the multidrug resistance class of transporters. This redistribution will be affected by the rapid turnover of phospholipids in the RBC membrane. The incorporation of new fatty acids at the inner monolayer and movement of these species to the outer monolayer links transbilayer movement with the network of proteins involved in the maintenance of the RBC phospholipid composition. The presence of ATP8A1 was reported in adult RBCs and the mRNA in RBC precursors, and confirmed by 2 recently published full-scale RBC proteome studies. When ATP8A1 is expressed in yeast vesicles, it acts as an aminophospholipid activated P-ATPase, which can be inhibited by similar factors that affect transbilayer movement in RBCs and is able to transport PS across a bilayer.
PS exposure in RBCs
The loss of the asymmetric distribution of phospholipids across the bilayer, and in particular the exposure of PS on the surface of the cell, is an important starting point in many physiologic processes, including the regulation of blood coagulation and the recognition and removal of apoptotic cells. Dying cells, with few exceptions, trigger cellular events that result in PS appearing on the cell surface. This PS exposure typically occurs during the last stage of the life of a cell, and triggers phagocyte docking and subsequent engulfment and degradation of the apoptotic cell. PS externalization is critical to this process, as its absence results in impaired recognition and clearance of apoptotic debris, which can lead to inflammatory and autoimmune responses. The appearance of PS at the apoptotic cell surface has been reported to be regulated by specific intracellular signaling events including mitochondrial stress, changes in cytosolic calcium, oxygen radicals, cytochrome c release, caspase activation, and DNA fragmentation. Similarly to apoptosis, PS is exposed in RBC when phospholipids are scrambled across the bilayer. Several in vitro conditions can lead to RBC PS exposure and PS-exposing RBCs in vivo are found in SCD. The underlying mechanism for this scrambling process and exposure of PS is not clear. It involves both a dysfunction of the flippase and increased transbilayer movement defined as phospholipid scrambling. Dielectric breakdown of the RBC membrane will increase transbilayer movement, and the incorporation of the calcium ionophore A23187 in the presence of Ca 2+ to scramble membrane phospholipids is well established. Exposure of PS on the surface induced by the increase in cytosolic calcium could involve a complex of phosphatidylinositol 4,5-bisphosphate (PIP 2 ) and Ca 2+ , but other factors have been shown to play a role. Ubiquitous RBC membrane proteins such as the anion exchanger band 3 (AE1) are reported to be involved in the movement of phospholipids across the membrane. Together, many factors may affect phospholipid movement across the RBC bilayer, and it has been difficult to clearly define a specific floppase or scramblase that is activated under conditions that lead to the exposure of PS.
PS exposure in SCD
In SCD and thalassemia, the exposure of PS on the RBC surface is highly amplified. In early studies, phospholipase treatment of RBC samples from sickle cell patients indicated a loss of phospholipid asymmetry related to the sickling process. It was thought that the separation of the membrane skeleton from the lipid bilayer in areas as the result of hemoglobin polymerization led to a rapid transbilayer movement and the exposure of PS in all sickled cells. Using flow cytometry and PS-labeling techniques, it has become clear that subpopulations of SRBCs expose PS. Most of the RBCs do not expose PS, and seem to be able to maintain their phospholipid asymmetry. Moreover, the size of the PS-exposing subpopulation varies largely from patient to patient. Although some patients do not seem to exhibit PS-exposing cells to a higher extent than controls, the presence of 10% of PS-exposing cells has been reported in the circulation of SCD patients.
The presence of these PS-exposing RBCs in the circulation may be a hallmark of premature aging of the RBCs and may be related to the anemia in these patients. The life span of RBCs in SCD is significantly shorter than the RBC survival in normal humans. Exposure of PS leads to recognition and removal of apoptotic cells in other tissues, and similarly, PS-exposing RBCs will be removed from the circulation. Interestingly these cells can be observed in the peripheral blood, thus indicating that PS-exposing RBCs are being generated in such high numbers that the normal processes to remove these PS-exposing cells are compromised, or simply unable to keep up. The compromised spleen function in sickle cell patients may play an important role in the inability of the body to remove these cells from the circulation. This finding seems underscored by the fact that PS-exposing cells are specifically found in thalassemia patients who are splenectomized. In addition to PS-exposing RBCs in the peripheral blood, RBC precursors are present in bone marrow, which, similar to nonviable cells in other tissues, trigger apoptotic processes. This process leads to the exposure of PS, and removal by macrophages. Particularly in thalassemia, erythropoiesis in bone marrow is marked by high levels of apoptosis or ineffective erythropoiesis. The presence of PS-exposing RBCs indicates the inability to maintain proper membrane organization, related to the network of phospholipid maintenance mechanisms as indicated earlier. Several of these processes occur simultaneously to maintain the proper phospholipid organization. Dysfunction of one or several of these mechanisms is, therefore, linked and can be related to the pathologic status. When RBCs from sickle cell patients are analyzed a large diversity in RBC density is observed, and SRBCs exhibit a wide range of PS externalization depending on the age and density of the cells, this density being correlated with a decreased rate of PS movement. The difference in density in subpopulations of SRBCs points to the inability of cells to maintain a proper ion balance across the membrane. Calcium-calmodulin was reported to be involved in PS exposure in SRBCs. Membrane ion transporters will be affected by the lipid organization of the membrane bilayer, which in turn may be linked to the altered phospholipid molecular species organization in these density-separated fractions. Because all RBCs are exposed to the same fatty acid substrates, a difference in phospholipid composition suggests a different phospholipid turnover, in turn suggesting alterations in the enzymes involved in the Lands pathway as described earlier. The exposure of PS on SRBC subpopulations is well established and the pathologic consequences of these cells are well recognized, although the underlying cause remains unclear. Initiation of scrambling by loading RBC with calcium leads ultimately to the exposure of PS, a process that is greatly accelerated when the cells are pretreated with sulfhydryl reagents such as N -ethylmaleimide (NEM). On the other hand, NEM treatment or oxidant damage inhibits flippase activity but does not lead to rapid PS exposure in vitro unless scrambling is also initiated. This finding suggests an efficient competition between the inward movement of PS and calcium-induced phospholipid scrambling, despite the rapid ATP decrease in the RBCs under these conditions and the inhibitory effect of calcium on the Mg-ATP–dependent ATP8A1. These data suggest that PS exposure becomes apparent in a short period of time only when both the inward movement is blocked and scrambling is initiated. The common denominator in PS-exposing SRBCs seems to be the inability to move PS from the outer to the inner monolayer. This apparent slower inward movement of PS can result from both a decreased inward movement of PS (lower activity of the aminophospholipid translocase) and a rapid scrambling (a return movement of PS from inner to outer monolayer). Measurements using intact RBC report on the transbilayer movement and distribution at steady state of the entire system, and not on the activity of a particular protein. In other words, these measurements describe the ability of the cell to maintain phospholipid asymmetry and do not describe the activity of a specific protein in the red cell membrane. Fluorescently labeled PC is not transported by an ATP-dependent mechanism. Hence a rapid redistribution of PC across the bilayer suggests increased phospholipid scrambling in PS-exposing cells. The measurement of movement of both fluorescently labeled PS and PC suggests that inward movement in SRBCs is inhibited and that this, together with an increase in scrambling, leads to PS exposure. As indicated earlier, both proteins and lipids are altered by oxidant damage during the life of the RBC. In normal RBCs thiol modification can alter transbilayer movement of aminophospholipid across the RBC bilayer, and similarly, movement is affected by oxidant stress. Normal or sickle murine RBCs that are temporarily depleted of ATP show a significant difference in their ability to restore PS asymmetry when ATP is restored, which is correlated with oxidant stress, suggesting that the flippase is irreversibly oxidatively damaged in subpopulations of SRBCs. Oxidant stress is random in nature, and many avenues may lead to an inhibition or alteration of the RBC flippase, ultimately leading to the inability of the RBC to maintain its phospholipid asymmetry. The mechanisms by which sulfhydryl reactive compounds and oxidant modifications act on the different isoforms of the ATP8A1 are poorly understood. The subpopulation of RBCs that exposes PS in SCD show an inhibited transbilayer movement of PS, and the inhibition by NEM points at 1 or more cysteines being vulnerable to oxidative damage, but other oxidative modifications cannot be excluded from playing a role in the inhibition of the flippase. ATP8A1 has 21 cysteines, all of which, based on sequence homology with other P-ATPases, are likely to be located in the RBC cytosol. It is currently not known which of these residues are involved in sulfhydryl bridges or are potentially vulnerable to modifications. In an intact RBC NEM affects many different proteins, and a direct relation between ATPase activity transport and NEM modification is difficult to make. At this point no direct proof is available on the oxidative modification of the flippase in SRBCs, in part because of the lack of detailed information on this protein, or the involvement (and dysregulation) of other components in the maintenance of phospholipid asymmetry. In a yeast vesicle system, the phosphorylation of the aspartate residue, essential to the phosphate cycle of P-ATPases, seems to be related to NEM modification of ATP8A1. Whereas both PS and PE are actively transported by ATP-dependent processes, RBC storage studies have shown that PE transbilayer movement in RBCs can be compromised while PS is still actively transported. These data suggest that inactivation of the flippase may not be an all-or-nothing process and, because 2 isoforms have been identified in RBCs, these forms may be differently affected. Sickle cell patients have a very diverse presentation of clinical pathology despite all of them having the same mutation in the β locus of hemoglobin. Similarly, the number of PS-exposing RBCs in the circulation varies significantly. The highly increased erythropoiesis may affect the expression of the different isoforms of ATP8A1. Several efforts are under way to link the clinical data in this patient population to differences in genetic background. These studies may also shed some light on the expression of ATP8A1 or the relevance of SNPs in the gene for ATP8A1. In sickle cell patients, specific SNPs have been identified as being related to the expression of HbF, in particular the repressor BCL11A, and/or to a positive response to hydroxyurea treatment. It cannot be excluded that abnormal regulation of erythroid-specific genes observed in SCD, including the modulation of treatment regimen aimed at gammaglobulin production, could also affect the expression of the isoforms of ATP8A1 as well as flippase activity or specificity.
Together the maintenance of composition and organization of the plasma membrane phospholipids are closely connected, and a loss of function in any of these components can be expected to lead to a loss of membrane viability and a relationship with the pathology of SCD.
Overall, a complex picture emerges of phospholipid movement, kinetics, and distribution for the different molecular species across the RBC bilayer. Nevertheless, the phospholipid headgroup distribution at equilibrium is well maintained during the life of the RBC.
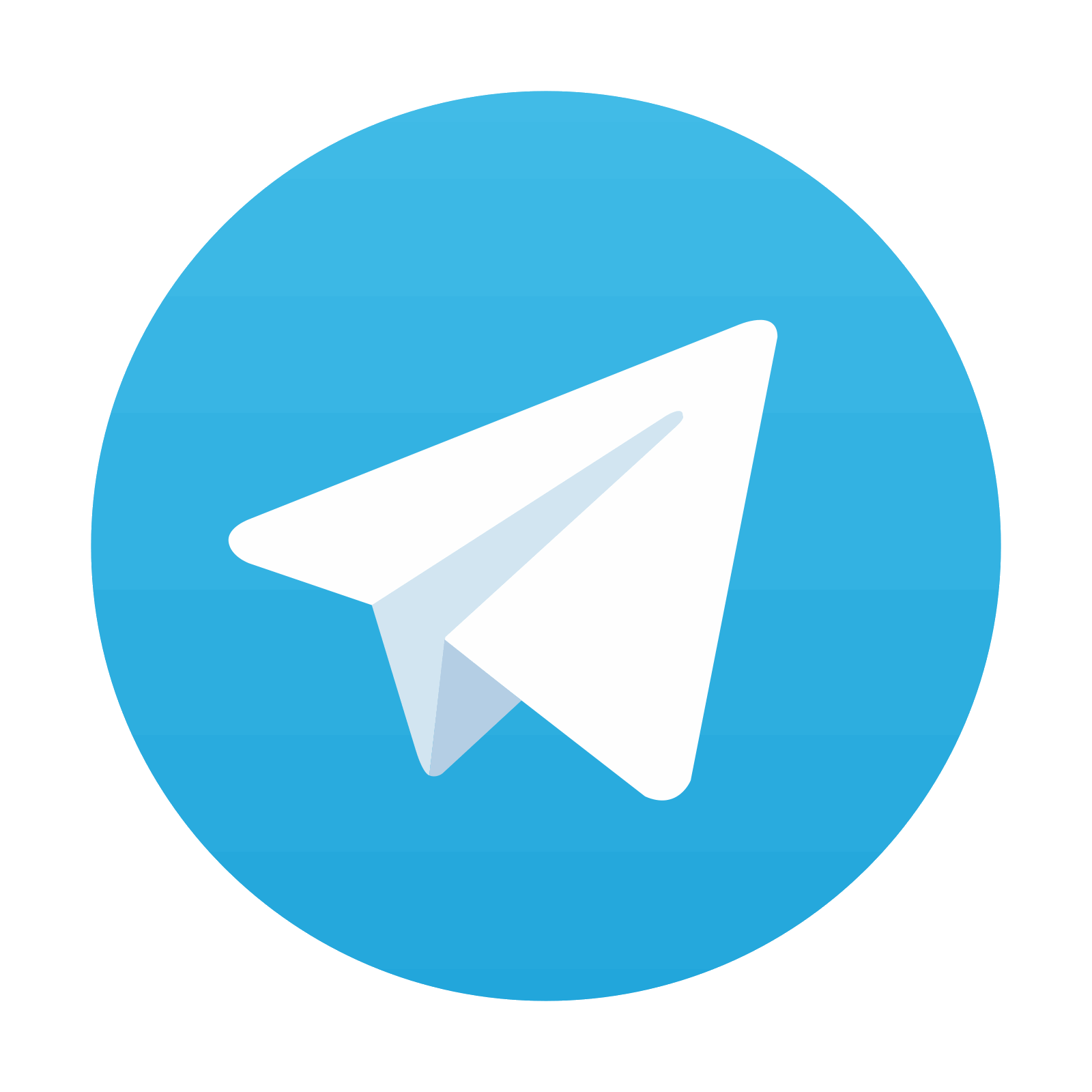
Stay updated, free articles. Join our Telegram channel
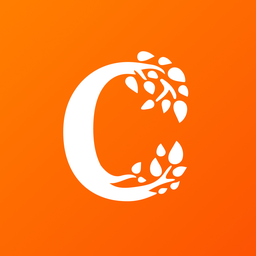
Full access? Get Clinical Tree
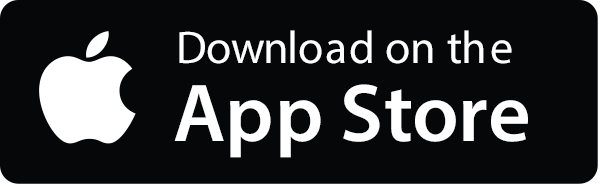
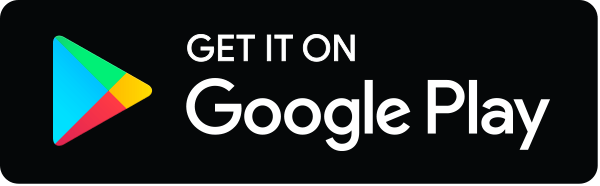