© Springer International Publishing AG 2018
Philip T. Cagle, Timothy Craig Allen, Mary Beth Beasley, Lucian R. Chirieac, Sanja Dacic, Alain C. Borczuk, Keith M. Kerr, Lynette M. Sholl, Bryce Portier and Eric H. Bernicker (eds.)Precision Molecular Pathology of Lung CancerMolecular Pathology Libraryhttps://doi.org/10.1007/978-3-319-62941-4_33. Genetic Susceptibility to Lung Cancer
(1)
Department of Pathology, University of Texas Medical Branch, Galveston, TX, USA
Keywords
Pulmonary adenocarcinomaLung cancerGenome-wide association studiesFamilial clusteringXenobioticsDNA adductsPolymorphismsLung Cancer in Nonsmokers
Most lung cancers are attributable to tobacco smoking, which exposes airways and lung parenchyma to numerous carcinogens and procarcinogens including free radical species, aromatic amines, polycyclic aromatic hydrocarbons, and nitrosamines. Compared to cigarette smoking, other exposures implicated in lung cancer impact lung cancer risk much less [1, 2]. Approximately 15% of lung cancers in men, and 50% of lung cancers in women, are not related to tobacco smoking [3, 4]. Overall, approximately 25% of lung cancer patients are never smokers [3, 4]. Although passive inhalation of tobacco smoke, also termed environmental tobacco smoke, is believed to play a role in some percentage of cases of lung cancer in never smokers [3], these tumors are usually designated idiopathic; and their histologic types differ from the types found in cigarette smokers [5]. Many of these never smokers who develop lung cancer are young women who develop adenocarcinoma and who show an overall better prognosis than patients with smoking-related lung cancers [6, 7]. Nonsmoking-related lung cancers are being increasingly recognized; and the disease likely represents a disease process unrelated to smoking-related lung cancer. People are thought to have variable susceptibilities to cancer risk factors, including lung cancer risk factors [8–38]. A genetic basis for differing cancer risk factor susceptibilities has been proposed based on the observation that different susceptibilities appear to be inherited based on aggregation of cancers within families [39–66]. Inherited susceptibilities would help explain why some people develop lung cancer, such as individuals with minimal or no tobacco smoke exposure [30, 36, 67–75], frequently in association with family histories positive for cancer [31, 75–79], or those who develop lung cancer from exposure at a significantly earlier-than-average age [80–87].
Complex gene-environment interactions occur, and genetic differences in susceptibility to tobacco smoke carcinogens exist; and lung cancers in both smokers and never smokers may have mutually common and distinct risk factors and gene-environment interactions [5]. Host susceptibility to lung cancer, individually or in synergy with smoking, is uncertain [88]; however, gene-environment interactions and genetic differences likely have a significant role in the development of lung cancer in never smokers [3, 86]. They also likely help explain why some heavy smokers do not develop lung cancer [3, 86, 89–92] and why some lung cancer patients have strong family histories of cancer [5, 93–96]. The human genome database and improving genotyping technology have substantially aided researchers in their search for and understanding of the genetic role of lung cancer development [3, 34, 86, 87, 91, 92, 97, 98].
Familial Clustering
Epidemiological studies suggest familial clustering of lung cancer occurs [3, 86, 87, 92]; the literature is in fact robust [60, 99–101]. Studies for which smoking exposure and occupational exposure were controlled have shown an increased risk of lung cancer in relatives of lung cancer patients [45, 49, 54, 59, 64]. Inherited polymorphisms in DNA repair genes and xenobiotic-metabolizing enzyme genes might account for the elevated risk, as might the genetic influence of substance dependence, including nicotine dependence [87, 102–107]. Multiple genetic loci may relate to nicotine dependence, including the promoter region of CHRNA5, a locus on chromosome 15 [108–110]. Studies of lung cancer patients’ families who were nonsmokers or significantly younger than average have shown an increased familial risk of lung cancer, supporting the premise that genetic susceptibility is a factor in lung cancer development [33, 34, 38, 47, 49, 59, 67–71, 76, 77, 80–82, 84, 87, 92, 101, 111].
A 2012 study identified a 1.25-fold risk increase for family history of lung cancer in nonsmokers who developed lung cancer [3, 112]. It is important to understand, however, that familial aggregation of lung cancer alone does not in and of itself prove inheritance of genetic risk variants [3]. Clustering of close relatives with similar exposures to environmental risk factors may exhibit itself as a familial aggregation. However, there is evidence that some situations exhibiting familial aggregation are the result of genetic variants [3].
Gender
Gender affects lung cancer incidence [85]. Female lung cancer patients who are never smokers are influenced by familial history than by radon gas or environmental tobacco smoke exposure [85]. Research is conflicting as to whether women smokers have an increased risk of developing cancer relative to men; however, some studies suggest women have an increased risk [113]. Some studies suggest that women smokers have an increased risk of developing lung cancer relative to men with the same smoking histories; however, other studies show women’s risk to be equivalent to men’s. Environmental factors, hormonal influences, and gender differences in xenobiotic-metabolizing enzymes are proposed reasons for reported differences in gender-associated lung cancer susceptibility.
Driver Genes
Epidermal growth factor receptor (EGFR) , echinoderm microtubule-associated protein-like 4-anaplastic lymphoma kinase (EML4-ALK) , proto-oncogene B-Raf (BRAF) , Kirsten rat sarcoma viral oncogene (KRAS) , and phosphatidylinositol-4,5-bisphosphate 3-kinase, catalytic subunit alpha (PIK3CA) are driver genes that may be mutated in pulmonary adenocarcinomas [114]. Gefitinib and erlotinib are EGFR tyrosine kinase inhibitors (EGFR-TKIs) used for molecular therapy of pulmonary adenocarcinomas [115]. EGFR-TKI-responsive EFGR mutations are identified more frequently in pulmonary adenocarcinomas of nonsmoking Asian women than in other groups of patients and are thought to arise early [116]. For example, one study of lung cancer patients showed EGFR mutations in 44% of pulmonary adenocarcinomas, with 47.5% occurring in women and 15% occurring in men and 42% occurring in nonsmokers and 14% occurring in smokers [117]. Nonsmoking Asian women whose pulmonary adenocarcinoma contains the EFFR mutation have been shown to have improved survival, particularly when the tumor demonstrates a significant lepidic pattern, compared to men with lung cancers who have a smoking history and whose pulmonary adenocarcinomas do not contain a significant lepidic pattern [2, 87, 118]. Pulmonary adenocarcinoma is identified relatively more frequently in nonsmoking women, and a relationship between EGFR mutation and membranous ERα expression has been identified as an independent prognostic factor in patients with pulmonary adenocarcinoma [116, 119]. HER2, a proto-oncogene of the receptor tyrosine kinase superfamily, binds other members of the EGFR family in the activation of EGFR signaling; and HER2 gene polymorphisms have been shown to increase susceptibility to pulmonary adenocarcinoma in nonsmoking Korean women [120].
Genome-Wide Association Studies
Genome-wide association studies (GWASs ) are population-level studies to identify genetic alleles associated with disease status or clinical phenotypes within the genome rather than in relation to a specific gene [85]. Lung cancer GWASs have shown several factors that correlate with lung cancer occurrence and progression [3, 85]. Several single-nucleotide polymorphisms (SNPs) in various genetic loci related to lung cancer susceptibility have been found; however, the three major susceptible loci associated with lung cancer risk are loci 15q24–25, 5p15, and 6p21 [121–124]. Locus 15p24–25 has been associated with lung cancer risk in Caucasian populations only, while loci 5p15 and 6p21 have been associated not only with lung cancer in Caucasian populations but also with lung cancer in East Asian (Korean, Japanese, Chinese) populations [123]. Other less well-established loci, including 3q28–29, 13q12.12, 22q12.2, and 18p11.22, have been identified as being associated with lung cancer in Asian populations [125].
Specific Polymorphisms Associated with Lung Cancer Susceptibility
Polymorphisms of xenobiotic-metabolizing genes and DNA repair genes have found potential allelic variants associated with lung cancer risk [126, 127]. The concept of polymorphisms of xenobiotic-metabolizing enzymes and DNA repair enzymes is appealing; however, studies correlating single-locus alleles with lung cancer risk have generally produced conflicting results, probably due to a number of factors. In some studies, the number of cases might be too few to reliably gauge the effects on lung cancer risk. Also, the polymorphisms studied might vary. Further, different ethnic groups exhibit widely differing frequencies of some polymorphisms, effecting results according to the ethnic group studied. Finally, as the metabolism, detoxification, and repair processes involved with DNA adducts are complex, one single polymorphism most likely does not account for differences in DNA adduct levels. Studies examining several or many polymorphisms simultaneously in a single population are more likely to yield more comprehensive and consistent results; and newer technologies, permitting the study of SNPs and haplotypes, increase statistical sensitivity [85].
Xenobiotic-Metabolizing Enzymes
Xenobiotics are drugs, toxins, solvents, and poisons, which are metabolized xenobiotic-metabolizing enzymes. Xenobiotics often induce xenobiotic-metabolizing enzymes by various methods, including by acting as substrate ligands that bind receptors, by activating the xenobiotic enzymes by transcription, or by stabilizing the protein product. Phase I xenobiotic-metabolizing enzymes metabolize the xenobiotic chemicals into other compounds; but paradoxically can metabolically bioactivate xenobiotic substrates, transforming them into active or more potent toxins or carcinogens, so-called reactive intermediates . The cytochrome P450s or CYPs are important phase I xenobiotic-metabolizing enzymes. Phase II enzymes detoxify reactive intermediates and transform them into compounds that can be removed from the body; the glutathione-S-transferases (GSTs) are an important class of phase II enzymes. Phase III transporters , including P-glycoprotein (P-gp), multidrug resistance-associated proteins (MRPs), and organic anion transporting polypeptide 2 (OATP2) are associated with xenobiotic transport and excretion [128–131].
Phase I enzymes P450s or CYPs primarily catalyze xenobiotic oxidation; however, they also catalyze reduction reactions. Also, CYPs are involved in other processes such as biosynthesis of steroid hormones and prostaglandins [129, 132, 133]. These reactions generally occur in the liver but can occur in other tissues, including lung tissue. 237–240 CYP-dependent metabolism often produces intermediate compounds called reactive intermediates that may be more potent carcinogens than their parent compounds and that could covalently bind to DNA and form adducts. DNA adduct formation is an important step in carcinogenesis. These intermediate compounds are also converted to more soluble, inactive products that may be excreted or compartmentalized by phase II enzyme-dependent conjugation reactions. CYP metabolism therefore may be a double-edged sword, leading to production of reactive intermediates that are more carcinogenic than the original compounds, but also more readily detoxified and removed than the original compounds. Nearly 60 active human P450 genes, mostly polymorphic, have been identified. CYP enzymes and genes are designated by family number (an Arabic number), subfamily letter (A, B, C, etc.), and individual members of a subfamily (also an Arabic number). Class I polymorphic CYP enzymes, which include CYP1A1, CYP1A2, CYP1B1, CYP2A6, CYP2E1, and CYP3A4, metabolize procarcinogens. CYP1A1 and CYP1B1 are particularly important for the metabolism of polycyclic aromatic hydrocarbons (PAHs) from tobacco smoke, and CYP2A6 and CYP2E1 are involved in the metabolism of nitrosamines from tobacco smoke [129, 131, 133, 134].
Many CYPs are induced by the aryl hydrocarbon receptor (AhR) , which acts by dimerizing with the AhR nuclear translocator (Arnt) and inducing expression of CYP1A1 and CYP1B1. CYP1A1 and CYP1B1 encode aryl hydrocarbon hydroxylases as well as CYP1A2. Ligands for AhR include PAHs and other xenobiotics which are also substrates for the activated CYP enzymes. AhR shows either low affinity or high affinity for its ligands, producing low or high inducibility of CYP1 enzymes. AhR, after binding its ligand, translocates into the nucleus and dimerizes with Arnt protein. The AhR/Arnt dimer then binds to xenobiotic responsive elements (XREs) of the CYP1A1 gene and activates its transcription [135, 136].
Benzo(a)pyrene is an extensively studied PAH found in tobacco smoke. It binds to AhR in the lungs, causing the induction of CYP1A1 and CYP1B1. CYP enzymes metabolically activate benzo(a)pyrene to benzo[a]pyrene-7,8-diol-9,10-epoxide (BPDE) . BPDE is a carcinogen that damages DNA by covalently bonding to the DNA, forming bulky chemical adducts, for example, by binding to guanine nucleobases in codons 157, 248, and 273 of p53—mutational “hotspots” in smoking-related lung cancers [137, 138]. Along with PAHs, tobacco smoke contains N-nitrosamines including 4-(methylnitrosoamino)-1-(3-pyridyl)-1-butanone (NNK), N-dimethylnitrosoamine (NDMA), N-diethylnitrosoamine (NDEA), N-nitrosophenylmethyl-amine (NMPhA), and N-nitrosonornicotine (NNN). These N-nitrosamines are metabolically activated by CYP2A6 and CYP2E1 to compounds that form chemical adducts with DNA [139, 140].
The phase II enzymes GSTs act mainly to catalyze the conjugation of glutathione (GSH) to xenobiotics containing an electrophilic center, forming more soluble, nontoxic peptides that are excreted or compartmentalized by other enzymes, the phase III enzymes. The GST superfamily is made up of enzymes that catalyze the conjunction of glutathione to xenobiotics and is divided into three subfamilies, each composed of multigene families—the soluble or cytosolic (canonical) GSTs, microsomal or MAPEG (membrane-associated proteins involved in eicosanoid and glutathione metabolism) GST, and plasmid-encoded bacterial fosfomycin-resistant GSTs. The cytosolic GSTs are polymorphic and make up seven classes—alpha, mu, and pi are regarded as specific and sigma, omega, theta, and zeta as common. Importantly, the cytosolic GSTs that assist in the metabolism of tobacco-derived carcinogens are GSTM1, GSTM3, and GSTP1 that detoxify reactive intermediates of PAHs such as benzo(a)pyrene and GSTT1 that detoxifies reactive oxidants such as ethylene oxide [141]. There are other phase II enzymes , including N-acetyltransferases (NAT), sulfotransferases (ST), UDP-glucuronosyltransferases (UGT), and NAD(P)H:quinone oxidoreductase (NQO1). Microsomal epoxide hydrolase (mEH) is a phase II enzyme which also acts as a phase I enzyme; it catalyzes the trans-addition of water to xenobiotics such as the PAH benzo(a)pyrene, producing dihydrodiol reactive intermediates involved in PAH-initiated carcinogenesis [141].
Xenobiotic-Metabolizing Genes
CYP Polymorphisms and Lung Cancer Susceptibility
Ayesh et al. suggested in 1984 that there was a relationship between lung cancer risk and a polymorphism of CYP (debrisoquine 4-hydroxylase or CYP2D6) [94]. Kawajiri et al. in 1990 proposed that CYP1A1 polymorphisms may impact on lung cancer risk [142]. Further research into CYP2D6 polymorphisms has produced mixed results [143–147]. Several CYP1A1 alleles have been extensively studied. The CYP1A1 m1 allele , also called MspI , has a T to C transition in the 3′ noncoding flanking region. It has increased enzyme activity. In 1991, Hayashi et al. described a transition of adenine to guanine at position 2455 in exon 7 of CYP1A1, causing an isoleucine to valine amino acid substitution at codon 462 (Ile462Val) [148]. Similar to the MspI allele, the valine allele or CYP1A1 m2 allele —also called CYP1A1*2C—has increased enzymatic activity (extensive metabolizer), thought to cause greater carcinogenic DNA adduct production and higher risk of tobacco smoke-related lung cancer. The CYP1A1 m3 allele , with a mutation in intron 7, is thought to be specific to African-Americans. The CYP1A1 m4 allele has a transition in exon 7 that causes a Thr for Asn substitution [148–154].
Several studies have explored the possible association between CYP1A1 polymorphisms and lung cance r risk in various ethnic populations [155–162]. CYP1A1 m1 and m2 polymorphisms strongly correlate with risk of lung cancer in several Japanese studies, especially with respect to tobacco smokers and squamous cell carcinoma of the lung [153, 163, 164]. Song et al. studied 217 Chinese lung cancer cases and 404 controls and identified an increased risk for pulmonary squamous cell carcinoma in patients with at least one CYP1A1 m1 allele or at least one CYP1A1 m2 allele [165]. Lin et al. has reported similar findings [166]. Persson et al. did not identify an association of lung cancer and CYP1A1 polymorphisms and Chinese patients who were predominantly women with adenocarcinomas [167].
As the prevalence of the CYP1A1 m1 and m2 alleles is extremely low in Caucasians, studies have generally exhibited mixed results regarding these polymorphisms and Caucasian patient lung cancer risk [168–170]. Le Marchand et al., studying pooled data from Caucasians from 11 studies with a total of 1153 lung cancer cases and 1449 control patients, identified an increased lung cancer risk, predominantly squamous cell carcinoma, associated with the presence of the CYP1A1 m2 allele [171]. Larsen et al., studying 1050 lung cancers and 581 controls, found an association between the CYP1A1 m2 allele and lung cancer risk, particularly among women patients, younger patients, and patients with lesser smoking histories [158]. Studies of populations of Americans with mixed ethnicity have also identified an increased lung cancer risk associated with the CYP1A1 m1 allele [169, 170]. Research from Brazil has also noted an increased risk of lung cancer associated with the CYP1A1 m2 allele [172, 173]. An increased risk for pulmonary adenocarcinoma, but not for other types of lung cancer, associated with the CYP1A1 m3 allele has been reported in African-Americans [174, 175].
New candidate genes have emerged in recent years as the percentage of pulmonary adenocarcinomas has increased, and the combination of CYP1A1*2B (Ile462Val) genotype and myeloperoxidase G/G (MPO G/G) genotype found to be associated with an increased risk of pulmonary adenocarcinoma. Increased risk in female never smokers has also been seen the slow genotype and the fast genotype among the polymorphisms of NAT2 [176]. Interestingly, as increased meat consumption may induce NAT2, lifestyle change may cause an increase in these xenobiotic gene activities [176, 177].
CYP2A6
CYP2A6 metabolically bioactivates N-nitrosamines in tobacco smoke [178]. Several alleles of CYP2A6 have been identified, including CYP2A6*4C, CYP2A6*7, CYP2A6*9, and CYP2A6*10. The alleles have decreased the enzyme activity or decreased expression of CYP2A6. These variant alleles of CYP2A6 are associated with a decreased lung cancer risk, especially for squamous cell carcinoma and small cell carcinoma, and a decreased risk in heavy smokers compared to light smokers and never smokers, a finding consistent with the decreased metabolic bioactivation of N-nitrosamines [178, 179].
Genetic variations in the CYPA6 nicotine metabolic gene and the CHRNA5-A3-A4 nicotine gene cluster have been found to be associated with increased risk of lung cancer. In addition to increased lung cancer risk, variation in CYP2A6 and CHRNA5-A3-A4 has been found to be associated with increased consumption of cigarettes and nicotine dependence [180]. Various studies have indicated that CYP2A6 deletions may be associated with increased risk of lung cancer [181–184].
GST and Lung Cancer Susceptibility
GST variants have been studied with respect to the risk of lung cancer, but the studies have yielded mixed results [185–190]. GST polymorphisms might also affect lung cancer cell type [191, 192]. These alleles occur in the GSTM1, GSTT1, GSTP1, and GSTM3 genes and are associated with the reduced activity or deletion, with loss of all activity, of these phase II enzymes. These alleles include the GSTM1*0 (GSTM1 null) allele, a deletion of the GSTM1 gene; the GSTT1*0 (GSTT1 null) allele, a deletion of the GSTT1 gene; the GSTP1 Ile105Val variant (I105V), caused by an A to G transition; the GSTP1 Ala114Val variant (A114V), caused by a C to T transition; and the GSTM3 intron 6 polymorphism, a three-base pair deletion in intron 6. Perera et al. found that adducts significant predicted lung cancer risk; that the combined GSTM1 null/GSTP1 Val genotype was associated with lung cancer generally, and especially in patients who were former smokers; and that adducts were significantly higher in patients who were current or former smokers with lung cancer who exhibited the GSTM1 non-null/GSTP1 Ile genotype [193]. In a meta-analysis of data from 130 studies containing 23,452 lung cancer cases and 30,397 controls, Ye et al. identified a weak association of the GSTM1 null and GSTT1 null polymorphisms with lung cancer risk and possibly weaker associations in studies of patients of European descent, whereas the GSTP1105V, GSTP1114V, and GSTM3 intron 6 polymorphisms showed no significant overall associations with lung cancer [190]. Hosgood et al. found that GST genotype GSTM1 null genotype may be associated with increased risk of lung cancer [194]. Okazaki et al. showed a relationship between nicotine dependence, indicated by increased serum nicotine levels, and CYP2A6 and CYP2B6 genes encoding nicotine-metabolizing enzymes and also CHRNB3 and CHRNA6 genes encoding nicotinic acetylcholine receptor subunits [195].
Other Phase II Xenobiotic Enzymes
Studies of NQO1 alleles and possible lung cancer risk have shown mixed results [196, 197]. Saldiver et al. noted that NQO1 variant allele associated with reduced activity was associated with increased lung cancer risk in younger patients, in women, and in never smokers [197]. Other authors studying NAT1 alleles and lung cancer risk have reached conflicting conclusions [198–200]. Habalova et al. identified a slow acetylation variant—*5B/*6—to be associated with squamous cell carcinoma risk in younger patients, in nonsmokers, and in women, whereas Wang et al. noted an increased risk of lung cancer in association with the SULT1A1*2 allele (variant A allele) which codes for a SULT1A1 sulfotransferase enzyme with decreased activity [200, 201].
Multiple Xenobiotic-Metabolizing Enzymes
Because xenobiotic metabolism is a complex process involving many enzymes, an accurate understanding of lung cancer susceptibility requires an understanding of the interactions of multiple genes and the effects of multiple enzymes. Several studies have examined the combined effects of two or more xenobiotic enzymes [202–205]. Hung et al., in a pooled analysis of data from 14 case-control studies that included 302 lung cancer cases and 1631 controls in Caucasian nonsmokers from the International Collaborative Study on Genetic Susceptibility to Environmental Carcinogens, identified an increased lung cancer risk with the combined CYP1A1 Ile462Val variant and GSTM1 null genotype relative to the CYP1A1 wild type and GSTM1 non-null genotype [203]. Raimondi et al. performed a meta-analysis of data from 21 case-control studies from the International Collaborative Study on Genetic Susceptibility to Environmental Carcinogens that included 2764 Caucasians, 555 lung cancer cases and 2209 controls, and 383 Asians, 113 lung cancer cases and 270 controls, who had never smoked on a regular basis [205]. Raimondi et al., in their analysis of multiple xenobiotic-metabolizing enzymes, found a significant association between risk of lung cancer and CYP1A1Ile462Val polymorphism in Caucasians, found GSTT1 deletion to be a lung cancer risk factor in Caucasian nonsmokers only, and found that the combination of CYP1A1 wild type , GSTM1 null , and GSTT1 non-null genotype s was associated with a lower risk of lung cancer. None of the polymorphisms examine studied by Raimondi et al. were associated with lung cancer in Asian nonsmokers [205].
New xenobiotic gene candidates have appeared as cases of pulmonary adenocarcinoma have increased. The CYP1A1*2B (Ile462Val) genotype with the myeloperoxidase G/G (MPO G/G) genotype displays a significantly increased pulmonary adenocarcinoma risk [159, 177]. Also, in nonsmoking women, the slow genotype and the fast genotype polymorphisms of NAT2 are related to lung adenocarcinoma risk; and lifestyle changes may increase these xenobiotic genes’ activities [176, 177].
DNA Adducts
DNA adducts from metabolically activated intermediates of compounds found in tobacco smoke are mutagenic and carcinogenic [206]. Bulky DNA adducts can be identified with 32P-postlabeling of tumor tissues, peripheral blood lymphocytes and other tissues, immunoassays and immunohistochemistry, mass spectrometry, fluorescence, HPLC electrochemical detection, and phosphorescence spectroscopy [207]. PAH-DNA adducts can be identified by BPDE-DNA immunoassays such as the BPDE-DNA chemiluminescence immunoassay (BPDE-DNA CIA); and elevated DNA adduct levels have been found in smokers’ lung and other tissues. More DNA adducts are found in patients with smoking-related cancers than in patients without cancer [208]. A meta-analysis showed that smokers with smoking-related cancers had a statistically significant (83% higher) level of DNA adducts than controls [209]. Increased levels of DNA adducts have been in smokers’ lungs relative to non- and never smokers’ lungs [210]. Along with studies demonstrating the carcinogenicity of DNA adducts from tobacco smoke, these studies support a link between DNA adduct number and lung cancer development. However, it must be remembered that in retrospective case-control studies, the possibility that the levels of DNA adducts are the result of, rather than the cause of, the disease cannot be completely excluded. Nonetheless, that DNA adducts are causative is strongly supported by prospective studies where DNA adducts were measured in blood samples collected years before cancer onset. One study showed that disease-free current smokers with elevated levels of DNA adducts in blood leukocytes were three times more likely to be diagnosed with lung cancer 1–13 years later than current smokers with lower DNA adduct levels [211]. Peluso et al. noted that the levels of leukocyte DNA adducts in blood samples collected several years before the onset of cancer were associated with the subsequent risk of lung cancer [212]. The association with lung cancer was stronger in never smokers—whose sources would be environmental, such as secondhand tobacco smoke and air pollution—and in younger patients. These prospective studies strongly support a relationship between DNA adduct levels and lung cancer risk. The studies also suggest that individual patients have differing susceptibilities to carcinogen exposures, highlighted by the risks observed in those with fewer years of exposure, younger patients, and those with lesser levels of exposure, never smokers.
DNA Repair Gene Polymorphisms
Because tobacco smoke contains carcinogenic chemicals which damage DNA, lung cancer has been shown to involve DNA damage repair genes, including O6-alkylguanine DNA alkyltransferase (AGT ) , X-ray repair cross-complementing group 1 (XRCC1 ) , NAD(P)H: quinoneoxidoreductase (NQO1 ) , human 8-oxoguanine DNA glycosylase (hOGG1 ) , cytosine DNA-methyltransferase-3B (DNMT3B ) , O6-methylguanine-DNA methyltransferase (MGMT ) , and several nucleotide excision repair (NER ) genes [195]. These gene polymorphisms inhibit the repair of damaged DNA, increasing the risk of carcinogenesis caused by tobacco [195]. In cultured lymphocytes, DNA repair capacity (DRC ) can be measured using the host-cell reactivation assay and a reporter gene damaged by the activated tobacco carcinogen BPDE. A fivefold variation in DRC has been found in the general population. Also, decreased DRC has been associated with increased lung cancer risk [213–216]. Polymorphisms in DNA repair genes may be related to differences in efficiency of DNA repair; and decreased or increased ability to repair DNA damage is thought to impact the accumulation of significant genetic abnormalities required for cancer development.
Prevalence of XPD alleles and genotypes varies greatly by ethnicity. Polymorphisms in codons 156, 312, 711, and 751 of the XPD gene are noted commonly, with an allele frequency greater than 20%. Polymorphisms of codon G23592A (Asp312Asn) of exon 10 and codon A35931C (Lys751Gln) of exon 23 cause amino acid changes in the XPD protein and have been studied with respect to lung cancer susceptibility [217–223]. Studies have examined the levels of DNA adducts associated with these polymorphisms as an indication of the efficiency of the different alleles at DNA repair. Most likely a higher level of adducts suggests that the allele has less efficiency at excising DNA adducts. With respect to codon 312 polymorphisms, most studies have found a higher level of DNA adducts in association with the Asn allele than with the Asp allele. The majority of studies have identified a higher level of DNA adducts in association with the Gln allele. As such, most studies indicate a difference in DNA repair efficiency between these specific XPD alleles [217, 221, 223, 224]. Hu et al., in a meta-analysis of data from nine case-control studies including 3725 lung cancer cases and 4152, found that patients with the XPD 751CC genotype have a 21% higher risk of lung cancer compared to patients with the XPD 751AA genotype and that patients with the XPD 312AA genotype have a 27% higher risk of lung cancer compared to the ones with the XPD 312GG genotype [224]. Performing a meta-analysis derived from the same studies as Hu et al., including 2886 lung cancer cases and 3085 controls for the XPD-312 polymorphism from 6 studies, and 3374 lung cancer cases and 3880 controls for the XPD-751 polymorphism from 7 studies, Benhamou and Sarasin were unable to conclude that one or the other of these polymorphisms was associated with an increased risk of lung cancer [224, 225]. After the conflicting meta-analyses, Hu et al. performed a case-control study that included 1010 lung cancer cases and 1011 age- and sex-matched cancer-free controls in a Chinese population [223]. Hu et al., studying eight SNPs/DIPs (deletion/insertion polymorphisms) of XPD/ERCC2 and XPB/ERCC3, found that none of the eight polymorphisms was individually associated with lung cancer risk; however, the combination of genetic variants in ERCC2 and ERCC3 contributed to the risk of lung cancer in a dose-response manner.
In other studies, an increased lung cancer risk with combinations of XPD polymorphisms and polymorphisms of other DNA repair genes has been identified [226]. Zhou et al. identified a significantly increased lung cancer risk in patients with five or six variant alleles of XPD Asp312Asn, XPD Lys751Gln, and XRCC1 Arg399Gln polymorphisms versus patients with no variant alleles [227]. Chen et al. found that patients with variant alleles for both XPD Lys751Gln and XRCC1 Arg194Trp polymorphisms have a higher lung cancer risk than patients with only one variant allele in a Chinese population [228].
Other DNA Repair Genes
Other DNA repair gene polymorphisms have received some examination with respect to lung cancer susceptibility, generally with conflicting or unconfirmed results, including the XPA [229, 230], XPC [231], XPG [232], XRCC1 [233, 234], XRCC3 [235], MMH/OGG1, BER pathway [236, 237], and MGMT [238]. Studying ATM genotypes in 616 lung cancer patients and 616 cancer-free controls, Kim et al. found that the A allele at the site (IVS62+60G>A) was associated with a higher lung cancer risk than the G allele [239]. Patients with the ATTA haplotype showed significantly increased lung cancer risk versus patients with the common GCCA haplotype; and patients with the (NN)TA haplotype showed an increased lung cancer risk versus patients without the (NN)TA haplotype.
Multiple DNA Repair Genes
Zienolddiny et al. found that (1) for the NER pathway , ERCC1 (Asn118Asn, C > T), ERCC1 (C15310G), and ERCC2 (Lys751Gln) variants were related to increased lung cancer risk and XPA, G23A, and ERCC5/XPG (His46His) variants were related to decreased lung cancer risk; (2) for the BER pathway , OGG1 (Ser326Cys) and PCNA (A1876G) variants were associated with increased lung cancer risk and APE1/APEX (Ile64Val) variant was associated with decreased lung cancer risk and variant T allele of PCNA2352 SNP had a marginal effect on cancer risk; (3) for the DSB-R pathway , XRCC2 (Arg188His) variant was related to increased lung cancer risk and XRCC9 (Thr297Ile) and ATR (Thr211Met) variants were associated with decreased lung cancer risk; and (4) for the DR pathway , MGMT/AGT (Leu84Phe) variant in exon 3 exhibited a slight tendency toward a higher lung cancer risk [161, 240]. Kiyohara et al. found that XPA G23A, OGG1, and ERCC2 polymorphisms were associated with increased risk of lung cancer [241].
TERT Polymorphism
Telomerase reverse transcriptase (TERT ) and cleft lip and palate transmembrane 1-like protein (CLPTM1L) , both on chromosome 5p15.33, have been reproducibly associated with lung cancer risk [121]. TERT encodes a telomerase subunit involved in maintenance of telomere ends. GWASs have identified chromosome 5p15.33 as a region related to risk of pulmonary adenocarcinoma [121]. Lung cancer risk has also been associated with SNPs in the 5p15.33 region [242]. TERT and CLPTM1L are two candidate susceptibility genes found on chromosome 5p15.33. Because TERT encodes a telomerase subunit important in the maintenance of telomere ends, its overexpression causes cellular life span prolongation. CLPTM1L, also termed cisplatin resistance-related protein 9, provides apoptosis resistance [243]. The rs2361000 SNP in the TERT gene has shown a strong association with lung cancer risk in never smokers; and an increased frequency of rs2736100 (TERT) as the risky allele has been seen in pulmonary adenocarcinoma, with the most significant association of rs2736100 at 5p15.33 in pulmonary adenocarcinoma in nonsmokers [242, 244, 245]. Besides 5q15.33, studies have shown no association at three additional susceptible loci at 10q25.2, 6q22.2, and 6q21.32 [245–247].
Conclusion
Genetic factors have clearly been shown to be related to susceptibility to lung cancer, particularly pulmonary adenocarcinoma. Genetic polymorphisms have been identified that have the potential to increase lung cancer risk. These genetic polymorphisms involve genes that are associated primarily with the metabolism of tobacco smoke carcinogens and the suppression of mutations induced by those carcinogens. Tobacco-associated versus nontobacco-associated lung cancers, gender differences, and geographic differences continue to be confounding factors in the evaluation of the genetic factors involved in lung cancer risk. Future studies likely will focus increasingly on the genetic factors related to smoking behavior and the psychological processes involved, even as studies continue to address biological predisposition for the development and progression of lung cancer [248].
References
1.
Higginson J, Jensen OM. Epidemiological review of lung cancer in man. IARC Sci Publ. 1977;1977:169–89.
2.
Clamon GH, Bossler AD, Abu Hejleh T, Furqan M. Germline mutations predisposing to non-small cell lung cancer. Familial Cancer. 2015;14:463–9.PubMed
3.
Musolf AM, Simpson CL, de Andrade M, Mandal D, Gaba C, Yang P, et al. Familial lung cancer: a brief history from the earliest work to the most recent studies. Genes. 2017;8:36.PubMedCentral
4.
Mitchell P, Mok T, Barraclough H, Strizek A, Lew R, van Kooten M. Smoking history as a predictive factor of treatment response in advanced non-small-cell lung cancer: a systematic review. Clin Lung Cancer. 2012;13:239–51.PubMed
5.
Gazdar AF, Boffetta P. A risky business—identifying susceptibility loci for lung cancer. J Natl Cancer Inst. 2010;102:920–3.PubMed
6.
Couraud S, Zalcman G, Milleron B, Morin F, Souquet PJ. Lung cancer in never smokers—a review. Eur J Cancer. 2012;48:1299–311.PubMed
7.
McCarthy WJ, Meza R, Jeon J, Moolgavkar SH. Lung cancer in never smokers: epidemiology and risk prediction models. Risk Anal. 2012;32(Suppl 1):S69–84.PubMedPubMedCentral
8.
Ikawa S, Uematsu F, Watanabe K, Kimpara T, Osada M, Hossain A, et al. Assessment of cancer susceptibility in humans by use of genetic polymorphisms in carcinogen metabolism. Pharmacogenetics. 1995;5 Spec No:S154–60
9.
Caporaso NE, Landi MT. Molecular epidemiology: a new perspective for the study of toxic exposures in man. A consideration of the influence of genetic susceptibility factors on risk in different lung cancer histologies. Med Lav. 1994;85:68–77.PubMed
10.
Braun MM, Caporaso NE, Page WF, Hoover RN. Genetic component of lung cancer: cohort study of twins. Lancet. 1994;344:440–3.PubMed
11.
el Zein R, Conforti-Froes N, Au WW. Interactions between genetic predisposition and environmental toxicants for development of lung cancer. Environ Mol Mutagen. 1997;30:196–204.PubMed
12.
Mooney LA, Bell DA, Santella RM, Van Bennekum AM, Ottman R, Paik M, et al. Contribution of genetic and nutritional factors to DNA damage in heavy smokers. Carcinogenesis. 1997;18:503–9.PubMed
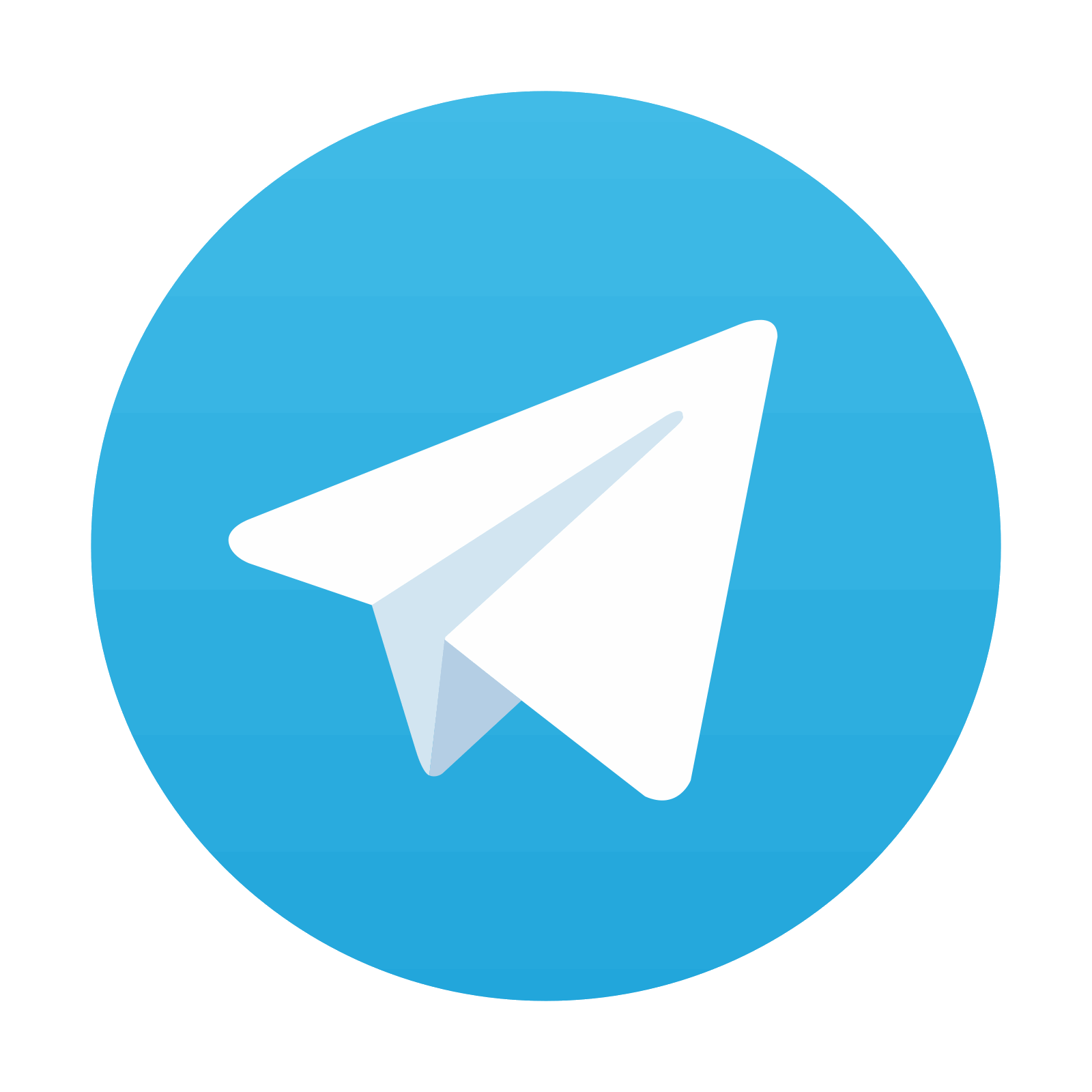
Stay updated, free articles. Join our Telegram channel
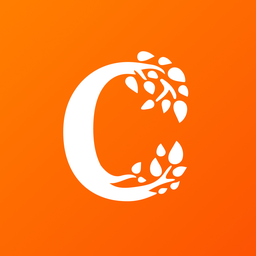
Full access? Get Clinical Tree
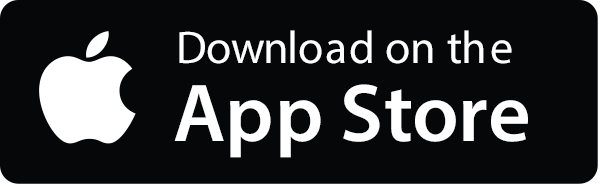
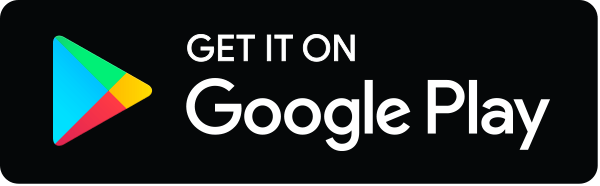
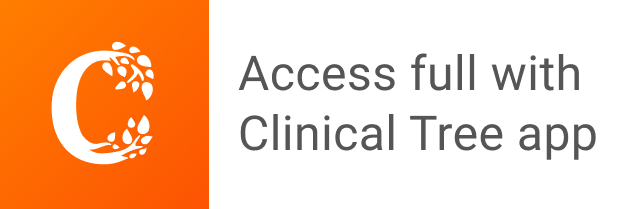