Fig. 14.1
Culture and differentiation of ME-LCs. hASCs were obtained from liposuction procedure. These cells were cultured along different stages in diverse media: DMEM-FBS (stage I), DMEM free-serum (stage II), SFO3 medium (stage III) and EC medium (stage IV). Cells obtained from stage III and stage IV were considered ME-LCs. These cells are capable to form capillary-like structures similar in comparison with the data rendered by HUVEC and differentiated to cardiomyocyte-like cells with the acquisition of a cardiogenic phenotype and the expression of cardiomyocyte-specific markers such as troponin T (red) and desmin (green)
Polymer Microarrays – Powerful Technology to Identify New Synthetic Matrices for Endothelial Progenitor Cells
Since the discovery of circulating ECs in peripheral blood a decade ago (Asahara et al. 1997), regenerative stem-cell-based therapeutic strategies have aimed at both developing novel therapies and improving current treatments by restoring ischemic tissues (Chan and Mooney 2008). Traditional methods of direct cell infusion or injection (which represent the most commonly utilized cell delivery strategy in the clinic – for example, BM transplants) often lead to big issues such as massive cell death, extremely poor homing/engraftment efficiency to targeted tissues and loss of control over the fate of transplanted cells (Karp and Leng Teo 2009). The introduction of biomaterial scaffolds as cell carriers provide a solution to these problems, due to their templating capability for guiding the formation of new tissue and for promoting engraftment within the host (Eschenhagen et al. 2012; Hench and Polak 2002). An emerging area of bioengineering research is, therefore, the development of synthetic biopolymer matrices as defined environments for EPC growth, providing sites of adhesion together with signals that can control EPC propagation and synchronize their differentiation (Mooney and Vandenburgh 2008).
This chapter describes a high throughput screening of polymer microarrays (Pernagallo and Diaz-Mochon 2011) for the identification of new polymer matrices which contain the necessary signals that not only promote adhesion and propagation of EPCs but also, synchronize their endothelial specialization. Due to the complexity and diversity of biocompatibility testing, it has been difficult to find a universal method which permits high throughput determination of in vitro properties. It is impossible to theoretically predict cellular response, and thus every time a new material is generated it is essential to test its biocompatibility using cells with which it will come into contact. To address this issue the development of a new system of screening, based on microarray technology has been developed. Polymer microarrays accelerate the process to find new biomaterials while minimizing expenditures regarding time and money. Furthermore, as these polymers are synthetic animal-free materials which can be manufactured to GMP standards they overcome one of the biggest issues, in terms of regulation and human health safety, of current industry standards, such as MatrigelTM, which have animal-source origins. Therefore, they can provide clinically useful materials for the construction of extra-corporeal devices and facilitate novel studies in modern vascular surgery.
Pre-formed and fully characterized polymer libraries were printed using a robotic printer onto agarose coated glass-slide following a defined xy pattern (Pernagallo and Diaz-Mochon 2011). These arrays were then used to identify new substrates for attachment, promotion, and propagation of EPCs which at the same time would keep cell functionality (Fig. 14.2 a, b).
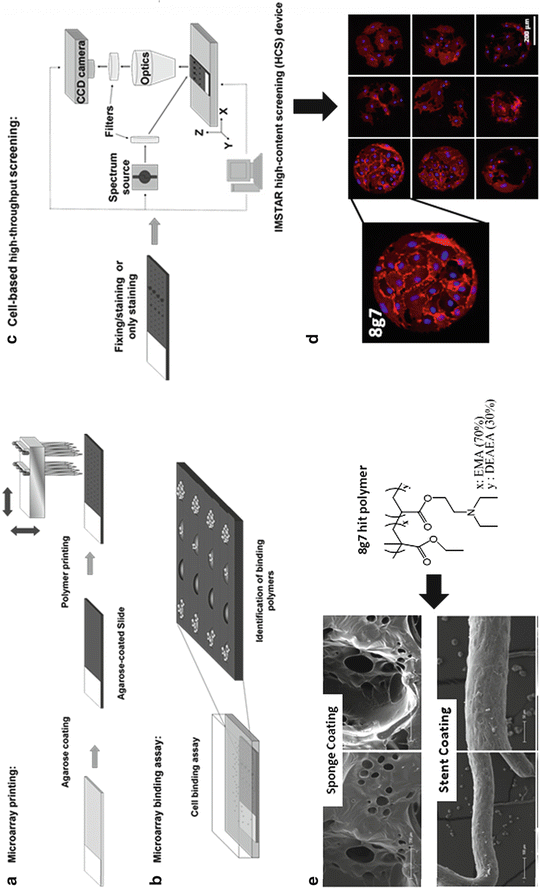
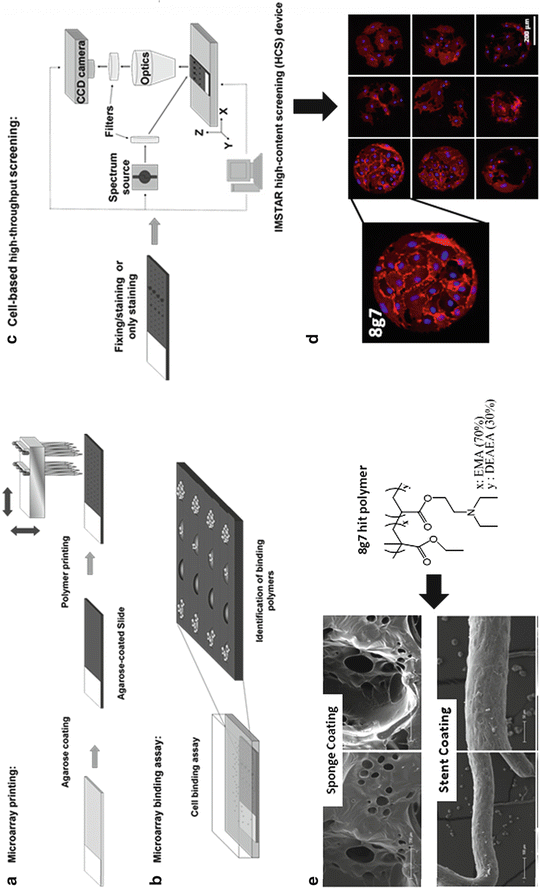
Fig. 14.2
Finding novel synthetic materials compatible with endothelial progenitors cells and their direct application for coating extra-corporeal devices. (a) Polymer microarray preparation; (b) cell-based microarray binding assay; (c) cell-based high-content screening; (d) fluorescence images from a polymer microarray incubated with EPCs and stained with Hoechst 33342 and CD31-PE antibody and (e) extra-corporal devices – sponges and stent – coated with a polymer – 8g7(see insert) – identified via polymer microarray approach (a–d) incubated with EPCs
Cord blood (CB) products (50 mL) were aspirated from umbilical placental veins from normal caesarean deliveries and collected into heparin (Pernagallo et al 2012). Mononuclear cells (MNCs) were isolated by buoyant density centrifugation and cultured over 3 weeks in endothelial basal medium-2 (EBM-2). By that time, spindle-shaped EPCs started to emerge. These cells express a number of endothelial markers comparable to a mature EC and human umbilical vein endothelial cells (HUVECs) and they are able to form new blood vessels in MatrigelTM (Skovseth et al. 2007). Thereafter cells were passaged until incubation with the polymer microarrays. As control HUVECs, which are specialized ECs, were also maintained in cell culture. At that point EPCs and HUVECs were removed from their substrate and plated onto two identical polymer microarrays containing 345 polyurethanes and polyacrylates, printed in quadruplicate given rise to 1,345 feature arrays. After 3 days of culturing, under standard cell culture conditions, cells were fixed and stained with a nuclear stain (Hoechst 33342), and conjugated monoclonal mouse anti-human CD31-PE antibody (Fig. 14.2c, d). CD31 (Anti-PECAM-1) recognizes the platelet/endothelial cell adhesion molecule-1 (PECAM-1), a 130–140-kDa single-chain integral membrane glycoprotein that is a member of the immunoglobulin gene superfamily (Pinter et al. 1997). The CD31 antigen is expressed on ECs, functions as a vascular cell adhesion molecule and is involved in the process of leukocyte migration through the intercellular junctions of vascular ECs (Delisser et al. 1997). Following fixing and staining, arrays were scanned using a High Content Screening Platform (Nikon 50i fluorescence microscope (×20 objective) with an automated XYZ stage, equipped with the PathfinderTM software package – IMSTAR) fit with two fluorescence channels (DAPI and Cy3). Cell numbers on spots were determined by analyzing images captured using DAPI channel (Hoechst 33342ex/em 355/465 nm) while endothelial phenotype was assessed via Cy3 channel (R-PE ex/em 545/575 nm).
Primary screening identified 6 polyurethanes and 12 polyacrylates that supported attachment of EPCs providing over 150 cells/mm2. After looking at initial data with more detail it was found out that 3 out of those initial 18 hits (3AA7, 8g7 and 9g7) provided to be excellent substrates, with over 200 cells/mm2, for both EPCs and HUVECs. These results supported the idea that 3AA7, 8g7 and 9g7, as well as enabling high cell adhesion could enhance endothelial maturation of EPCs towards specialized cells such as HUVECs. In order to study the endothelial function in further detail and confirm the results of these experiments, the three selected polymers (3AA7, 8g7 and 9g7) were scaled-up and spin-coated onto glass cover slips which were then used to grow both EPCs and HUVECs for flow cytometry analysis. EPCs and HUVECs cultured on polymer – coated surfaces, expressed endothelial cells surface markers vWF, VEGFR2 (also referred to as Flk-1 or KDR), endothelial nitric oxide, synthase (eNOS), CD31, CD34, CD105 and CD146 but not the hematopoietic cell surface antigens CD45. Therefore, flow cytometry results confirmed HTS data, thus demonstrating that new substrates, identified via polymer microarray approach, enhance specialization of EPCs (Pernagallo et al. 2012).
Generation of Coated Devices to Make Them Biocompatible with Endothelial Progenitor Cells
Further studies were undertaken by cultivating EPCs on pieces of sponges coated with a solution of 8g7 (Pernagallo et al. 2012). The ultimate aim of studying sponge as a cell scaffold was to generate functional 3-D tissues outside of the organism, which might then be implanted into the organism. In an ideal scenario 8g7 coated sponges would have the ability to adhere EPCs in a in vitro fashion before being implanted into animals so that they can either promote the growth and the specialization of those cells previously anchored to the sponge as well as recruit distant BM-derived progenitor cells for restoring vascularization at the implanted scaffold/host tissue interface. One cm3 sponges were dip-coated in a 1% w/w solution of 8g7 in THF and further sponges were also dip-coated in the current gold standard, Growth Factor Reduced MatrigelTM (GFR-MG). Polymer coated sponge showed a smoother uniformly coated layer allowing a friendlier environment for cells to grow. The coated sponge was impregnated with either EPCs or HUVECs in complete EBM-2 medium that supports endothelial cultivation. After overnight incubation, cells were fixed and SEM analysis carried out. SEM images clearly showed that only sponges pre-coated with polymer allowed tube formation while sponges pre-coated with GFR-MG lacked tube formation features (Fig. 14.2d). It was also clear that by increasing number of EPCs adhered to the 8g7 sponge, a major release of both cytoprotective and pro-angiogenic factors in a paracrine manner to promote the survival and proliferation of endothelial cells happened (Gnecchi et al. 2008). The functional 3-D tissue generated in vitro was tested for its ability to promote cell growth and recruit distant BM-derived progenitor cells for promoting engraftment with the host, after implantation. Therefore, sponges were pre-treated with 8g7 and loaded with EPCs in vitro. Following initial incubation, these sponges were implanted into animals. Each animal had a EPCs-loaded 8g7 impregnated sponges on one side and a control EPCs loaded native sponge on the other. Twenty days after implantation, mice were sacrificed and sponges were excised and analyzed for vessel formation. Sponges were divided into three pieces and analyzed as follows: SEM imaging; Chalkley count and hemoglobin assay (Pernagallo et al. 2012).
After implantation in mice, the sponges impregnated with 8g7 promoted better engraftment within the host, showing clearly the formation of a vascular network in contrast with the blank (uncoated) sponge. Notably, uncoated sponge resulted in a poor and incomplete engraftment within the host, showing the native sponge without cells at several points. It seems evident that the large number of EPCs adhered to the 8g7 sponge, allowed not only a large quantity of local cell growth but also made possible a major release of cytokines which recruited distant BM-derived progenitor cells that effectively mediated vascularization at the implanted scaffold/host tissue interface. Histological analysis revealed that significantly increased numbers of vessels formed on the polymer coated sponge compared with the control (sponge uncoated). One part of the sponge was fixed in 10% formalin and embedded in paraffin. Sections (5 μm) were stained with haemaoxylin/eosin for identification of blood vessels. Sponge vessel density was determined by using the mean of triplicate Chalkley counts on two sections per sponge. Histology showed vascularization by a number of blood vessels but in polymer coated sponges had significantly more blood vessels compared to non polymer coated sponges. A further study focused on measuring hemoglobin levels of the sample. This is an excellent method of determining the frequency of blood vessels, as they are proportional to the blood hemoglobin concentration. Polymer coated sponges had significantly more hemoglobin, displaying ~twofold increase compared to uncoated polymer sponges.
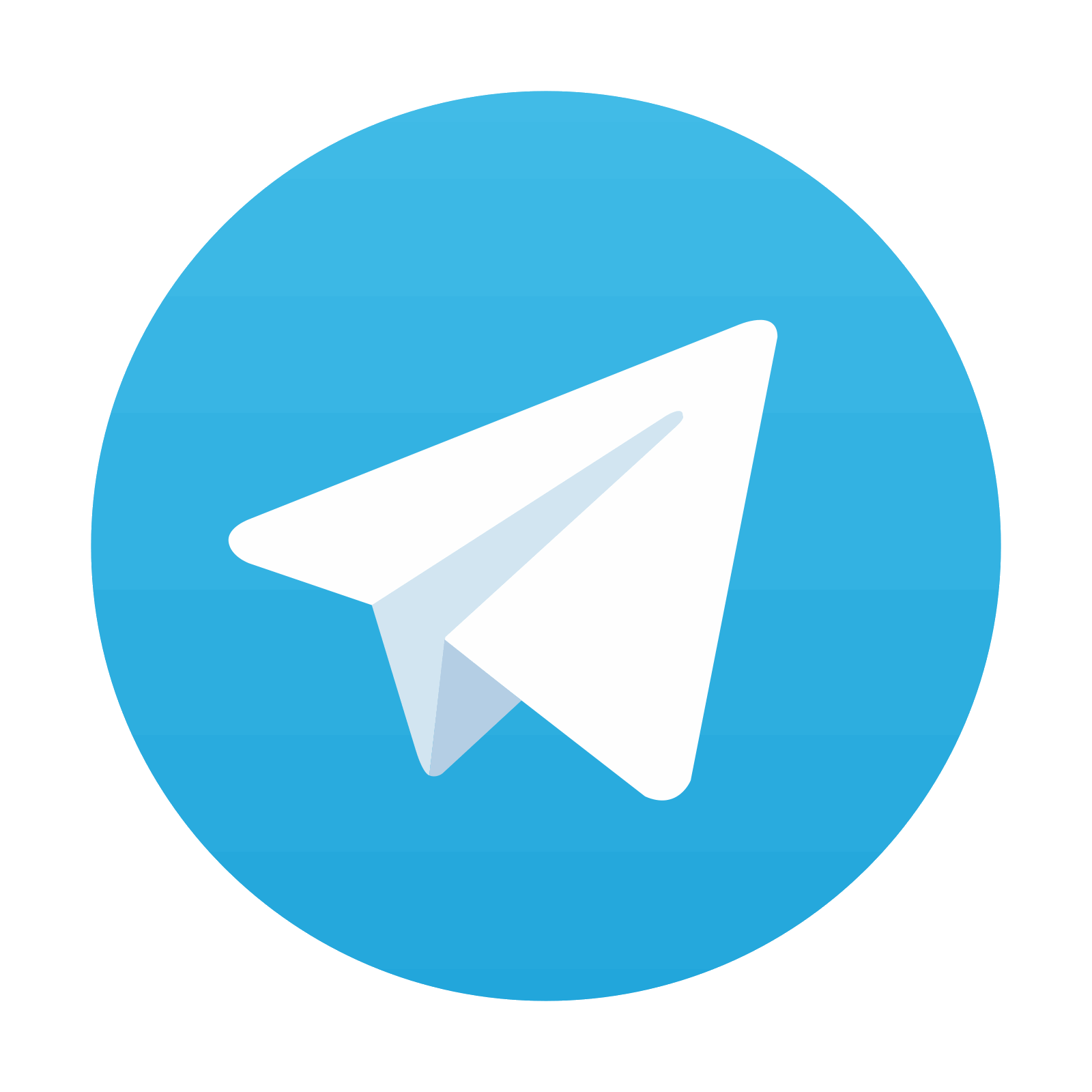
Stay updated, free articles. Join our Telegram channel
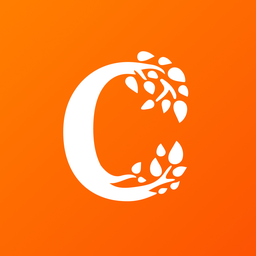
Full access? Get Clinical Tree
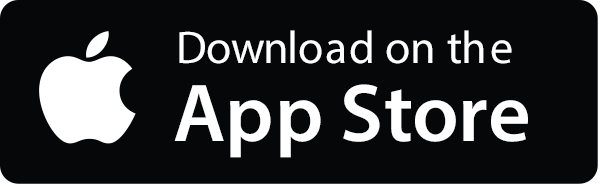
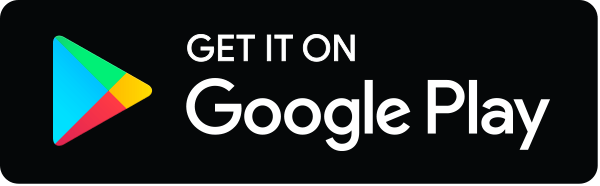