Keywords
Radiotherapy, Radioprotection, MnSOD-PL
Introduction: The Radiotherapy Update
Radiation therapy (radiotherapy) remains one of the three critical approaches toward the management of the cancer patient, along with surgery and chemotherapy . There are two general categories in the approach toward delivering radiotherapy to cancer cell volumes: brachytherapy (radioactive source implant procedures) and external beam radiotherapy . Brachytherapy involves the application of permanent radiation sources (radium and cesium) using intracavitary or mold application and also interstitial application (using needles, wire, or catheter placement with later temporary insertion of radiotherapy low-dose-rate or high-dose-rate sources) delivered as radiation to localize tissue with the highest dose and dose rate close to each source and the falloff of dose with the square of the distance in centimeters from the source. Techniques of brachytherapy designed during the first half of the 20th century greatly improved the clinical outcomes of many cancer patients, primarily those with head and neck cancer and gynecological malignancies including cervix cancer and endometrial cancer . Both acute and late effects of brachytherapy relate to the total dose delivered, dose rate, and concomitant surgical procedures required to insert and remove sources .
In brachytherapy, radioactive source removal has been alleviated by the use of permanent radioactive seed implantation techniques and by the use of short-lived radioisotopes to deliver localized dose that then decays over days to weeks to radiation levels that are low or undetectable, leaving seeds in place within the tumor requiring no further surgical procedure for removal, which is different from the surgical staple technique.
In contrast to brachytherapy, external beam radiotherapy utilizes focused aiming of one or multiple radiation beams each culminated to combined shape the radiation dose around a tumor volume. The first kilovoltage (ortho-voltage) radiotherapy machines utilized diagnostic X-ray machines , the use of which was often associated with severe skin reactions due to backscatter of low-energy photon energy from the calculated tumor volume depth back to the skin. The advent of the cobalt-60 teletherapy unit in the mid-20th century and modern linear accelerators facilitated dose deposition deep in the tissues with minimal skin reaction . High linear energy transfer particle beam radiotherapy, including protons, neutrons, and, recently, carbon atom accelerators, further facilitated precise focus of irradiation dose deep into tissues with a relative decrease in normal tissue dose. Finally, a sophisticated computer-driven X-ray (photon) delivery system utilizing moving collimators, moving treatment machine gantries, and techniques of image-guided radiotherapy (in which computed tomography (CT) scans or diagnostic-quality X-rays are matched to the radiotherapy beam) allows focus delivery of multiple photon beam radiotherapy . The toxicity to normal tissues is determined by the number of radiotherapy fractions (number of treatments during 6 or 7 weeks), dose delivered per treatment, overall treatment time and days, and volume of normal tissue treated.
By the mid-20th century, parameters for safe delivery of therapeutic irradiation using either brachytherapy or external beam radiotherapy had been well-established. The advent of combination chemotherapy, using increasingly effective cytotoxic drugs and, recently, biological response modifiers, has required modification of the “safe” parameters for radiation therapy because normal tissue toxicity increased with the improvement in tumor control . The therapeutic setting is even further complicated by the wealth of new date from positron emission tomography/CT scanning and sophisticated cancer cell imaging techniques, which have allowed identification of microscopic disease in both adjacent and distant locations from the primary tumor. This necessitates discussions of how large a volume of tissue to treat and whether the focus should be on stereotactic radiosurgery (high dose, high dose rate, and pencil-diameter beam delivery to nodal areas) .
Finally, use of total body irradiation to prepare leukemia, multiple myeloma, and other solid tumor patients for bone marrow transplantation or for uses with additional “systemic” chemotherapy agents provides the ultimate challenge to radiation oncologists with respect to improving the therapeutic ratio . In the case of total body irradiation, shielding of normal tissues such as lung with transmission block fractionating irradiation or lowering the dose rate can decrease toxicity, but they also increase the likelihood of tumor recurrence .
Gene Therapy Approaches to Enhance Management of the Radiotherapy Patient
The discovery of oncogenes and tumor suppressor genes and the identification of gene translocation specifically within tumor cells provide a challenge to adapt gene therapy techniques to cancer patients. Accordingly, the first gene therapy strategies targeted tumor cells in an attempt to counterbalance or overload abnormal cancer cell-specific gene expression with “normal” gene expression. In the case of p53 , gene deletion, or blocked expression of gene product, lung cancer patients provided a logical target for insertion of wild-type p53 transgene. Furthermore, lung cancer tumor localization by diagnostic radiographs and transgene insertion through stereotactic thoracic surgical techniques facilitated successful clinical trials . The same strategy held for head and neck cancers found to contain mutated epidermal growth factor receptors (EGFRs), facilitating a clinical trial in which insertion of wild-type EGFR localized surgical injection techniques followed .
Therapeutic transgene application to tumor targets also included approaches in which an additive or synergistic cytotoxic agent could be inserted by localized surgical technique. Prostate cancer target volume treated with herpes simplex virus-activated cytotoxin was found to be of therapeutic benefit combined with external beam radiotherapy. Ionizing irradiation-activated promoters linked to transgenes provided an ideal method by which to localize transgene activation in tumor volumes within the radiotherapy beam.
Although all tumor-specific gene therapy approaches provided attractive model systems, and many were successfully translated to the clinic, a common problem was the difficulty in finding a vector to facilitate transgene expression in a significant number of tumor cells within a target volume . Other concerns were the role of tumor vasculature , the role of supportive stromal cells , and the effect of infiltrating immune cells in both the response to the transgene product and its interaction with ionizing irradiation . Gene therapy successes in targeting vascular structures in other noncancer diseases gave encouragement to the field. Localized injection of transgene into tumor volume necessitated localization of tumor, targeting with insertion devices, and the attendant trauma associated with localized gene delivery. This strategy was most obvious with head and neck and lung cancer and brain tumors . Hemorrhage was a risk and could have resulted from inserting needles to allow localized expression of transgene . Irradiation late effects, primarily fibrosis or scarring, were a concern for possible deleterious additive toxic effects of gene delivery to tumors . An alternative approach of systemic (intravenous) delivery of transgene, while avoiding localized traumatic insertion techniques, created the new problem of transgene expression in surrounding tissues within the radiotherapy target volume or distant sites . A significant concern was the possible higher level of expression of a radiosensitizing transgene product in normal tissues compared to tumor—a situation that could tip the therapeutic ratio in the wrong direction, producing later radiotherapy toxicity . Finally, the use of fractionated irradiation in external beam approaches and the limited degree of expression of the transgene necessitated multiple transgene administrations , which were impractical in cases of surgical operative locations for injection to sustain bioavailability of gene product.
With all of the concerns for potentially critical toxicities, new approaches have focused on the identification of transgene products that would be more toxic to cancer cells compared to normal tissues. For example, in the case of improved tumor cell survival under hypoxic conditions, delivery of transgene products specifically toxic to hypoxic cells (HIF1 inhibitor), presumably nontoxic to normal tissues, might be a strategy for tumor-specific transgene targeting. Figure 9.1 shows the normal tissue of the lung separate from a lung cancer. The insert shows tumor cells, blood vessel endothelial cells, stromal cells, immune cells, and the problem of targeting the tumor for transgene expression.
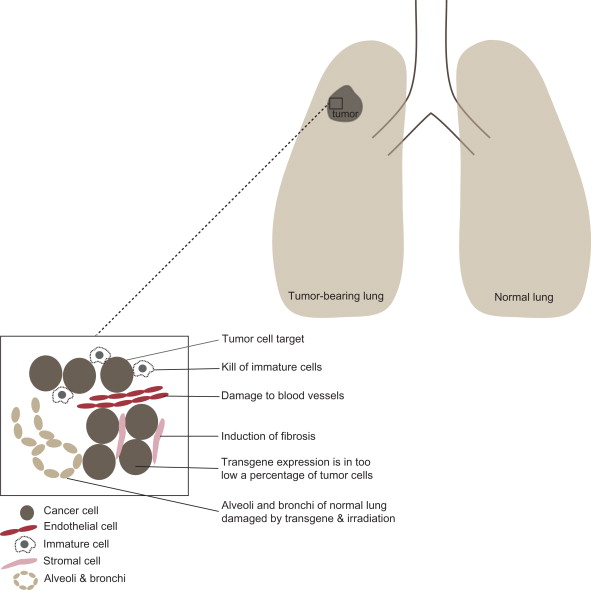
Normal Tissue Radioprotective Gene Therapy: The Logic of this Reverse Strategy
Since the initial use of radiotherapy in the management of cancer patients, normal tissue side effects have been of great importance in choosing radiotherapy dose, beam energy, and daily fraction size . Symptoms of radiation toxicity are treated with palliative measures until a therapeutic (tumoricidal) dose is achieved or the intensity of radiotherapy side effects prevents completion of the treatment plan. The best approach toward normal tissue protection has been, and continues to be, the blocking of normal tissues by precise radiotherapy beam columniation. There has been replacement of 10 half-value layer lead blocks with movable multileaf collimators .
The concept of radioprotective gene therapy for normal tissues followed studies that designed small molecule normal tissue radioprotectors . Studies during the 1970s at the Walter Reed Research Institute led to the discovery of WR2721 , a free radical scavenger compound now therapeutically known as amifostine . Systemic and organ-specific delivery of the radioprotector amifostine showed some positive therapeutic effects. Most prominent has been the clear demonstration of protection of the salivary glands and reduction of xerostomia in head and neck cancer patients receiving amifostine , attributable to the high concentration of drug in salivary glands. Attempts to use locally applied amifostine to protect rectal tissue to increase the efficiency of radiotherapy of prostate cancer, or swallowed amifostine to treat the esophagus or inhaled or systemic drug to protect normal lung, have had less therapeutic success. The strategy of normal tissue radioprotection using small molecules led to the concept that gene therapy could also be used for normal tissue protection and offered the added potential advantage of transient—but perhaps lasting several days—continuous production of transgene transcript and protein that is perhaps more effective and long enough in duration for clinical radioprotection .
Identification of targets for normal tissue radioprotection using gene therapy originated with an analysis of the components of the normal tissue response to irradiation. Figure 9.2 shows potential targets for development of a transgene therapy for radioprotection of normal tissues (DNA strand breaks, nucleus-to-mitochondrial targeting, apoptosis, cell death, cytokine elaboration, and secondary cell death). Strategies by which to prepare transgenes targeting DNA repair, to lessen DNA strand breaks, were difficult due to the nonspecificity of transgene uptake in normal compared to tumor tissue. Strategies to target cytokine production by irradiation-damaged cells to prevent secondary normal tissue apoptosis were also difficult because they needed to avoid tumor radioprotection. A promising strategy was that associated with gene therapy approaches to target the oxidative stress response of normal tissue compared to cancer cells to ionizing irradiation, specifically the apoptotic cellular pathway .
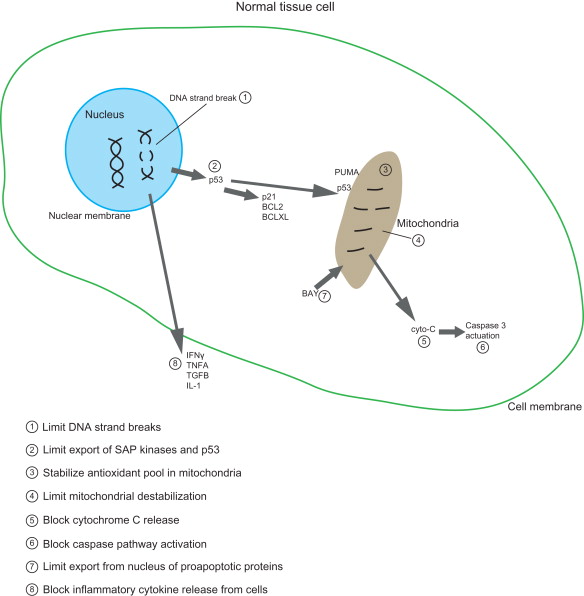
Pioneering studies by Biaglow et al . and Bump et al . demonstrated that the ionizing irradiation-induced DNA strand breaks were followed by rapid depletion of antioxidant stores, prominently glutathione, within cells. Irradiation also induced high levels of antioxidant enzymes , including the superoxide dismutases . A major advancement in the understanding of radiation-induced cell death in normal tissues was the identification of the role of communication between nuclear DNA strand breaks and mitochondrial mechanisms of apoptosis . Irradiation-induced DNA strand breaks are followed immediately by phosphorylation of the ATM protein and establishment of a DNA repair complex at the site of strand breaks involving genes within the Fanconi pathway and those associated with homologous recombination and nonhomologous end joining . Following DNA strand breaks and initiation of DNA repair, proteins are translocated from nucleus to mitochondria, including those of the stress-activated protein kinase pathway , JNK1, p38, as well as cell cycle regulatory proteins p53 and p21 . The mitochondrial membrane interaction of pro-apoptotic (BAX) and anti-apoptotic (BCLXL and BCL2) proteins establishes a balance that is tipped either toward ion channel stabilization to limit mitochondrial membrane permeability and stabilize the mitochondrial membrane or toward destabilization . Protein interactions altering the mitochondrial membrane occur simultaneously with changes in free radical production . Prominent in the cascade of radiation-induced free radicals is the interaction between superoxide and nitric oxide . The combination of superoxide with nitric oxide forms peroxynitrite, a potent initiator of lipid peroxidation .
Data indicate that stable interaction between cytochrome c and cardiolipin within the mitochondrial membrane is critical to stabilize the mitochondria from initiating apoptosis . Oxidative stress at the mitochondria produces multiple negative and destabilizing changes in the antioxidant pool . One change is the conversion of cytochrome c into a peroxidase that oxidizes cardiolipin and facilitates its separation from cytochrome c. Cytochrome c then releases from the mitochondrial membrane during oxidative stress-induced changes and initiates activation of the caspase system and apoptosis . Another change induced by oxidative stress in the mitochondria is the nitration of superoxide dismutase (SOD), which converts this antioxidant enzyme into a peroxidase . Oxidized SOD, even in a tetrameric form, continues to neutralize superoxide, whereas the nitrated form destabilizes the antioxidant pool . Knowledge of free radical biochemistry at the mitochondria and mitochondrial membrane presented multiple targets for potential gene therapy approaches for radioprotection of normal tissues .
Practical Applications: MnSOD-Plasmid Liposome Gene Therapy
The concept of using an antioxidant transgene for radioprotection of normal tissues derives from an understanding of the normal tissue radiation response. Pioneering studies by multiple groups, including those led by Philip Rubin , Lawrence Marks , and Michael Anscher , demonstrated that irradiation of normal tissue upregulates a series of proteins, including several termed inflammatory cytokines and “early response genes.” Upregulation of interleukin-1, tumor necrosis factor-α, transforming growth factor-β, and interferon-γ was demonstrated to occur both in tissues within irradiated fields and in peripheral blood cells and distant sites. Further studies demonstrated that other categories of gene transcripts and gene products were also elevated in the immediate minutes to hours after ionizing irradiation exposure . Some of these gene products in the irradiation response were common to other oxidative stress pathways, including ischemia reperfusion injury, hyperthermia, hypoxia, and response to chemotherapeutic toxic agents .
One category of genes upregulated in the ionizing irradiation response was that of the cellular oxidative stress response . Prominent among the genes associated with the oxidative response was manganese superoxide dismutase . MnSOD (SOD2) is one of three superoxide dismutases functioning to neutralize superoxide, a free radical induced by ionizing irradiation. Unlike cytosolic SOD1 and extracellular SOD3, MnSOD (SOD2) has a mitochondrial targeting peptide that localizes the protein to the mitochondrial membrane . Initial studies transfecting hematopoietic progenitor cell line 32D cl 3 demonstrated that SOD2, in its intact form but not in a form deleting the mitochondrial targeting sequence, significantly increased radioresistance of cells in culture . In reciprocal experiments, Cu/ZnSOD (SOD1) was ineffective as a radioprotector, but when linked to the mitochondrial localization sequence of MnSOD, it became a potent radioprotector . These in vitro studies led to translation of the use of MnSOD transgene therapy to clinical radiation protection.
Initial radioprotection studies of MnSOD transgene used plasmid liposomes (PL) and adenovirus for radioprotection of the lung . Due to the inflammatory response to adenovirus , the less toxic PL approach was taken. Amelioration of late radiation fibrosis/organizing alveolitis in the C57BL/6J mouse model demonstrated that intratracheal injection or inhalation of MnSOD-PL using an aerosol in a nebulizer device produced significant uptake of epitope tagged MnSOD in alveolar type II cells, pulmonary alveolar macrophages, and endothelial cells of the lung . In contrast, there was significantly less uptake of MnSOD transgene and product expression in orthotopic tumors in the mediastinum or lung, and there was no significant radiation protection of these orthotopic tumors .
Reports by Oberley et al . and St. Clair et al . indicated that squamous cell tumors originating in several different anatomic sites demonstrated a downregulation of MnSOD due to mutation in the promoter region or other defects in transcription and translation. The downregulation of MnSOD in tumors conferred an inability of these tumor cells to biochemically degrade hydrogen peroxide, the product of MnSOD dismutation of irradiation-induced superoxide , because peroxidase was also downregulated. Consistent with these observations was a report that glutathione peroxidase , the biochemical mediator of H 2 O 2 metabolism to water, was downregulated in human tumors. In this study, Oberley et al . demonstrated that transfection of tumor cells with a transgene for MnSOD produced hydrogen peroxide that was toxic to tumor cells and had no toxic effect on normal tissue cells that had an intact MnSOD pathway. Transfection of a glutathione peroxidase transgene (to mediate biochemical degradation of hydrogen peroxide to water) along with the MnSOD transgene reversed the toxicity of hydrogen peroxide in tumor cells .
Data on orthotopic lung tumors and head and neck cancers confirmed and extended the observations by Oberley et al . demonstrating that local administration of MnSOD-PL significantly protected the normal lung, esophagus, oral cavity, tongue, and oropharynx while having significantly less effect on orthotopic tumors. The therapeutic effect was mediated by a difference in antioxidant pool size in normal tissues compared to tumors and also by the hypersensitivity of tumor cells to hydrogen peroxide in comparison to normal tissues . The successful application of intratracheal or inhalation delivery of MnSOD-PL gene therapy led to studies confirming the effectiveness of the MnSOD transgene as a radioprotector for esophagus , oral cavity and oral mucosa , bladder , intestine , pregnant mice , bone wound healing , and total body irradiation (TBI) . In TBI studies, MnSOD transgene, delivered by plasmid liposomes, was shown to localize in the liver as well as other tissues and to confer significant radioprotection against both the acute and the chronic effects (life shortening) of TBI . When added to an antioxidant diet in the post-irradiation period, further improvement in late effects of TBI (decreased life shortening) was observed .
The previously mentioned studies demonstrated the potential effectiveness of antioxidant gene therapy in the treatment of squamous cell tumors and also in the normal tissues from TBI.
Future Directions in and Applications of Gene Therapy in Radiation Oncology
What would be the ideal gene therapy application in radiation oncology? Several investigators have suggested one that would combine the following five principles of gene therapy:
- 1.
Use of targeting transgene to the tissue/organ of interest with gene expression in an adequate number of cells to provide therapeutic effect in the setting of ionizing irradiation
- 2.
Minimal toxicity for normal tissues
- 3.
Safety of the methodology, meaning rapid elimination of the transgene product from normal tissues with no delayed deleterious effect on somatic cells or germ cells, no transmission of gene product through the germline, and effective elimination of the gene product with death of tumor cells
- 4.
In the setting of tumor radiosensitization, activation of the transgene product specifically in tumors by ionizing irradiation directly or indirectly through the radiation-induced bystander effect
- 5.
In the setting of normal tissue radioprotective gene therapy, utilization of a transgene product that would have no protective effect on tumor cells and ideally could provide simultaneous tumor radiosensitization
Two groups of investigators have published clever approaches to achieve some or all of these goals . There have since been successes both in animal studies and in clinical trials .
Future research in gene therapy approaches to radiotherapy will benefit from the availability of new and safe vectors for therapeutic use, including lentiviral vectors for transducing hematopoietic cells in tumors and “safe” non inflammatory adenovirus vectors , adeno-associated viral vectors , and the availability of tissue-specific lipid formulations and emulsions to deliver transgenes . All these studies have advanced the field of gene therapy. Future successes will be dependent not only on the safety of new therapeutics but also on further understanding of tumor biology. Advances in understanding the oncogenic process in specific tumors, including identification of EGFR receptor mutations in lung cancer, K-Ras-overexpressing adenocarcinomas, tumor categories with specific gene deletions, chromosome translocations, and changes altering cell membrane physiology including redox balance, have provided targets not only for small-molecule therapeutics but also for gene therapy approaches.
With respect to the use of radiotherapy in managing primary tumors in close anatomic proximity to critical organs (lung cancer located near heart and spinal cord, spinal cord tumors, esophageal cancer, brain tumors, pancreas cancer, and others), new insight has been gained from studies of the tumor microenvironment. Primary cancers contain not only malignant cancer cells (including quiescent cancer stem cells and differentiated progeny) but also a network of stromal cells, endothelial cells, and inflammatory response cells including macrophages, subsets of T lymphocytes, actual killer cells, and mononuclear leukocytes ( Figure 9.1 ). Innovative gene therapy approaches include using cells of the tumor microenvironment to carry transgenes into the tumor. Granulocyte macrophage colony-stimulating factor transgene expressing cells injected into the tumor stimulate leukocytes to migrate to the tumor. Also, strategies that use gene therapy to limit growth of the cellular support elements of the tumor microenvironment are examples of new approaches. Limiting tumor neovasculature growth by endothelial cell targeting and using growth factor-producing bone marrow stromal cells (mesenchymal stem cells) to carry transgenes into tumors to limit tumor growth or increase the tumor response to ionizing irradiation are two other ideas.
The disciplines involved in cancer therapy now have new novel approaches for combined modality therapy including gene therapy. Further studies will be required to define the ideal gene therapy targeting system to optimize the outcomes of radiotherapy of cancer patients.
References
- [1]. DeVita V.T., Lawrence T.S., Rosenberg S.A., DePinino R.A., and Weinberg R.A.: Cancer: principles & practices of oncology. Philadelphia: Lippincott Williams & Wilkins, 2011.
- [2]. Tysome J.R., Wang P., Alusi G., Briat A., Gangeswaran R., Wang J., et al: Lister vaccine strain of vaccinia virus armed with the endostatin-angiostatin fusion gene: an oncolytic virus superior to . Hum Gene Ther 2011; 22: pp. 1101-1108
- [3]. Bradley K.A., and Petereit D.G.: Radiation therapy for gynecologic malignancies. Hematol Oncol Clin North Am 2006; 20: pp. 347-361
- [4]. Hall E.J., and Giaccia A.J.: Radiobiology for the radiologist. Philadelphia: Lippincott Williams & Wilkins, 2006.
- [5]. Thirion P., Holmberg O., Collins C.D., O’Shea C., Moriarty M., Pomeroy M., et al: Escalated dose for non-small-cell lung cancer with accelerated hypofractionated three-dimensional conformal radiation therapy. Int J Radiat Oncol Biol Phys 2004; 71:
- [6]. Epperly M.W., Franicola D., Zhang X., Nie S., and Greenberger J.S.: Effect of EGFR receptor antagonists gefitinib (Iressa) and C225 (Cetuximab) on MnSOD-plasmid liposome transgene radiosensitization of a murine squamous cell carcinoma cell line. In Vivo 2006; 20: pp. 791-796
- [7]. Pan J.-J., Zhang S.-W., Chen C.-B., Xiao S.-W., Sun Y., Liu C.-Q., et al: Effect of recombinant adenovirus-p53 combined with radiotherapy on long-term prognosis of advanced nasopharyngeal carcinoma. J Clin Oncol 2009; 27: pp. 799-804
- [8]. Seow Y., and Wood M.J.: Biological gene delivery vehicles: beyond viral vectors. Mol Ther 2009; 17: pp. 767-777
- [9]. Lai S.Y., Koppikar P., Thomas S.M., Childs E., Egloff A.M., Seethala R., et al: Intratumoral EGFR antisense DNA therapy in head and neck cancer: first human application and potential antitumor mechanisms. J Clinical Oncology 2009; 27: pp. 1235-1242
- [10]. Grandis J.R., Melhem M.F., Gooding W.E., Day R., Holst V.A., Wagener M.M., et al: Tumor levels of TGF-α and EGFR protein predict survival in patients with neck squamous cell carcinoma. J Natl Cancer Inst 1998; 90: pp. 824-832
- [11]. He Y., Zeng Q., Drenning S.D., Melhem M.F., Tweardy D.J., Huang L., et al: Inhibition of human squamous cell carcinoma growth . J Natl Cancer Inst 1998; 90: pp. 1080-1087
- [12]. Freytag S., Stricker H., Movsas B., Elshaikh M., Aref I., Barton K., et al: Gene therapy of prostate cancer. In Roth J.A., (eds): Gene-Based therapies for cancer. New York: Springer, 2010.
- [13]. Freytag S., Stricker H., Pegg J., Paielli D., Pradhan D., Peabody J., et al: Phase I study of replication-competent adenovirus-mediated double suicide gene therapy in combination with conventional dose three-dimensional conformal radiation therapy for the treatment of newly-diagnosed, intermediate-to high-risk prostate cancer. Cancer Res 2003; 63: pp. 7497-7506
- [14]. Freytag S., Stricker H., Peabody J., Pegg J., Paielli D., Movsas B., et al: Five-year followup of trial of replication-competent adenovirus-mediated suicide gene therapy for treatment of prostate cancer. Mol Ther 2007; 15: pp. 636-642
- [15]. Freytag S., Movsas B., Aref I., Stricker H., Peabody J., Pegg J., et al: Phase I trial of replication-competent adenovirus-mediated suicide gene therapy with IMRT for prostate cancer. Mol Ther 2007; 15: pp. 1016-1023
- [16]. Barton K., Stricker H., Brown S., Elshaikh M., Aref I., Lu M., et al: Phase I study of noninvasive imaging of adenovirus-mediated gene expression in the human prostate. Mol Ther 2008; 16: pp. 1761-1769
- [17]. Freytag S., Stricker H., Movsas B., and Kim J.H.: Prostate cancer gene therapy clinical trials. Mol Ther 2007; 15: pp. 1042-1052
- [18]. Freytag S., Barton K., Zhang Y., Paielli D., Tyson D., Nall C., et al: Replication-competent adenovirus-mediated suicide gene therapy with radiation in a preclinical model of pancreatic cancer. Mol Ther 2007; 15: pp. 1600-1606
- [19]. Freytag S., Khil M., Stricker H., Peabody J., Menon M., DePeralta-Venturina M., et al: Phase I study of replication-competent adenovirus-mediated double suicide gene therapy for the treatment of locally recurrent prostate cancer. Cancer Res 2002; 62: pp. 4968-4976
- [20]. Yan S.F., Pinsky D.J., Mackman N., and Stern D.M.: Egr-1: is it always immediate and early? J Clin Invest 2000; 105: pp. 553-554
- [21]. Gilmore T.D.: Introduction to NF-KB: players, pathways, perspectives. Oncogene 2006; 25: pp. 6680-6684
- [22]. Keeney P.M., Quigley C.K., Dunham L.D., Papageorge C.M., Iyer S., Thomas R.R., et al: Mitochondrial gene therapy augments mitochondrial physiology in a Parkinson’s disease cell model. Hum Gene Ther 2009; 20: pp. 897-907
- [23]. Wolfe D., Wechuck J.B., Krisky D.M., Goff J.P., Goins W.F., Ozuer A., et al: Delivery of herpes simplex virus-based vectors to stem cells. Methods Mol Biol 2004; 246: pp. 339-352
- [24]. Wilber A., Hargrove P.W., Kim Y.-S., Riberdy J.M., Sankaran V.G., Papanikolaou E., et al: Therapeutic levels of fetal hemoglobin in erythroid progeny of β-thalassemic CD34 . Blood 2011; 117: pp. 2817-2821
- [25]. Trobridge G.D., Allen J., Peterson L., Ironside C., Russell D.W., and Kiem H.-P.: Foamy and lentiviral vectors transducer canine long-term repopulating cells at similar efficiency. Hum Gene Ther 2009; 20: pp. 519-523
- [26]. Cooper M., Nayak S., Hoffman B.E., Terhorst C., Cao O., and Herzog R.W.: Improved induction of immune tolerance to factor IX by hepatic AAV-8 gene transfer. Hum Gene Ther 2009; 20: pp. 767-776
- [27]. Li H.L., Zheng X.Z., Wang H.P., Li F., Wu Y., and Du L.F.: Ultrasound-targeted microbubble destruction enhances AAV-mediated gene transfection in human RPE cells . Gene Ther 2009; 16: pp. 1146-1153
- [28]. Chawla S.P., Chua V.S., Fernandez L., Quon D., Saralou A., Blackwelder W.C., et al: Phase I/II and phase II studies of targeted gene delivery . Mol Ther 2009; 17: pp. 1651-1657
- [29]. Rajendran S., O’Hanlon D., Morrissey D., O’Donovan T., O’Sullivan G.C., and Tangney M.: Preclinical evaluation of gene delivery methods for the treatment of loco-regional disease in breast cancer. Exp Biol Med 2011; 236: pp. 423-434
- [30]. Chiocca E.A., Aguilar L.K., Bell S.D., Kaur B., Hardcastle J., Cavaliere R., et al: Phase IB study of gene-mediated cytotoxic immunotherapy adjuvant to up-front surgery and intensive timing radiation for malignant glioma. J Clin Oncol 2011; 29: pp. 3611-3619
- [31]. Ho I.A.W., Miao L., Sia K.C., Wang G.Y., Hui K.M., and Lam P.Y.P.: Targeting human glioma cells using HSV-1 amplicon peptide display vector. Gene Ther 2010; 17: pp. 250-260
- [32]. Dronadula N., Du L., Flynn R., Buckler J., Kho J., Jiang Z., et al: Construction of a novel expression cassette for increasing transgene expression . Gene Ther 2011; 18: pp. 501-508
- [33]. Gardlik R., Behuliak M., Palffy R., Celec P., and Li C.J.: Gene therapy for cancer: bacteria-mediated anti-angiogenesis therapy. Gene Ther 2011; 18: pp. 425-431
- [34]. Zibert J.R., Wallbrecht K., Schon M., Mir L.M., Jacobsen G.K., Trochon-Joseph V., et al: Halting angiogenesis by non-viral somatic gene therapy alleviates psoriasis and murine psoriasiform skin lesions. J Clin Invest 2011; 121: pp. 410-420
- [35]. Samaranayake H., Maatta A.-M., Pikkarainen J., and Yla-Herttuala S.: Future prospects and challenges of antiangiogenic cancer gene therapy. Hum Gene Ther 2010; 21: pp. 381-396
- [36]. Shen J.K., Yang X., Xie B., Chen Y., Swaim M., Hackett S.F., et al: MicroRNAs regulate ocular neovascularization. Mol Ther 2008; 16: pp. 1208-1216
- [37]. Ke B., Shen X.-D., Gao F., Qiao B., Ji H., Busuttil R.W., et al: Small interfering RNA targeting heme oxygenase-1 (HO-1) reinforces liver apoptosis induced by ischemia-reperfusion injury in mice: HO-1 is necessary for cytoprotection. Hum Gene Ther 2009; 20: pp. 1133-1142
- [38]. Manning E., Pham S., Li S., Vazquez-Padron R.I., Mathew J., Ruiz P., et al: Interleukin-10 delivery via mesenchymal stem cells: a novel gene therapy approach to prevent lung ischemia-reperfusion injury. Hum Gene Ther 2010; 21: pp. 713-727
- [39]. Katz M.G., Swain JaBaris D., White J.D., Low D., Stedman H., and Bridges C.R.: Cardiac gene therapy: optimization of gene delivery techniques . Hum Gene Ther 2010; 21: pp. 371-380
- [40]. Griffin M.D., Ritter T., and Mahon B.P.: Immunological aspects of allogeneic mesenchymal stem cell therapies. Hum Gene Ther 2010; 21: pp. 1641-1655
- [41]. Bainbridge J.W.B., Smith A.J., Barker S.S., Robbie S., Henderson R., Balaggan K., et al: Effect of gene therapy on visual function in Leber’s congenital amaurosis. N Engl J Med 2008; 358: pp. 2231-2239
- [42]. Cideciyan A.V., Hauswirth W.W., Aleman T.S., Kaushai S., Schwartz S.B., Boye S.L., et al: Human RPE65 gene therapy for Leber congenital amaurosis: persistence of early visual improvements and safety at 1 year. Hum Gene Ther 2009; 20: pp. 999-1004
- [43]. Lavazza C., Carlo-Stella C., Giacomini A., Cleris L., Righi M., Sia D., et al: Human CD34 . Blood 2010; 115: pp. 2231-2239
- [44]. Candolfi M., Xiong W., Yagiz K., Liu C., Muhammad A.K.M.G., Puntel M., et al: Gene therapy-mediated delivery of targeted cytotoxins for glioma therapeutics. Proc Natl Acad Sci USA 2010; 107: pp. 20021-20026
- [45]. Li W., Zhang L., Johnson J.S., Zhijian W., Grieger J.C., Ping-Jie X., et al: Generation of novel AAV variants by directed evolution for improved CFTR delivery to human ciliated airway epithelium. Mol Ther 2009; 17: pp. 2067-2077
- [46]. Yan W., Wang P., Zhao C.X., Tang J., Xiao X., and Wang D.W.: Decorin gene delivery inhibits cardiac fibrosis in spontaneously hypertensive rats by modulation of transforming growth factor-β/Smad and p38 mitogen-activated protein kinase signaling pathways. Hum Gene Ther 2009; 20: pp. 1190-1200
- [47]. Waddington S.N., Crossley R., Sheard V., Howe S.J., Buckley S.M.K., Coughlan L., et al: Gene delivery of a mutant TGFβ3 reduces markers of scar tissue formation after cutaneous wounding. Mol Ther 2010; 18: pp. 2104-2111
- [48]. Marrotte E.J., Chen D.-D., Hakim J.S., and Chen A.F.: Manganese superoxide dismutase expression in endothelial progenitor cells accelerates wound healing in diabetic mice. J Clin Invest 2010; 120: pp. 4207
- [49]. Gau P., Rodriguez S., DeLeonardis C., Chen P., and Lin D.M.: Air-assisted intranasal instillation enhances adenoviral delivery to the olfactory epithelium and respiratory tract. Gene Ther 2011; 18: pp. 432-436
- [50]. Movsas B., Scott C., Langer C., Werner-Wasik M., Nicolaou N., Komaki R., et al: Randomized trial of Amifostine in locally advanced non-small cell lung cancer patients receiving chemotherapy and hyperfractionated radiation: radiation therapy oncology group trial 98-01. J Clin Oncol 2005; 23: pp. 2145-2154
- [51]. Spence A.M., Krohn K.A., Edmondson S.W., Steele J.E., and Rasey J.S.: Radioprotection in rat spinal cord with WR-2721 following cerebral lateral intraventricular injection. Int J Radiat Oncol Biol Phys 1986; 12: pp. 1479-1482
- [52]. Greenberger J.S.: Radioprotection. In Vivo 2009; 23: pp. 323-336
- [53]. Greenberger J.S., Epperly M.W., Gretton J., Jefferson M., Nie S., Bernarding M., et al: Radioprotective gene therapy. Curr Gene Ther 2003; 3: pp. 183-195
- [54]. Greenberger J.S., and Epperly M.W.: Radioprotective antioxidant gene therapy: potential mechanisms of action. Gene Ther Mol Biol 2004; 8: pp. 31-44
- [55]. Greenberger J.S., and Epperly M.W.: Pleiotrophic stem cell and tissue effects of ionizing irradiation protection by MnSOD-plasmid liposome gene therapy. In Columbus F. (eds): Progress in gene therapy. Hauppauge, NY: Nova Science, 2005. pp. 110-118
- [56]. Epperly M.W., Melendez , Zhang X., Nie S., Pearce L., Peterson J., et al: Mitochondrial targeting of a catalase transgene product by plasmid liposomes increases radioresistance . Radiat Res 2009; 171: pp. 588-595
- [57]. Biaglow J.E., Lee I., Donahue J., Held K., and Tuttle S.: Glutathione depletion or radiation treatment alters respiration and induces apoptosis in R3230Ac mammary carcinoma. Adv Exp Med Biol 2003; 530: pp. 153-164
- [58]. Bump E.A., Yu N.Y., and Brown J.M.: Radiosensitization of hypoxic tumour cells by depletion of intracellular glutathione. Science 1982; 217: pp. 544-545
- [59]. Epperly M.W., Bray J.A., Kraeger S., Zwacka R., Engelhardt J., Travis E., et al: Prevention of late effects of irradiation lung damage by manganese superoxide dismutase gene therapy. Gene Ther 1998; 5: pp. 196-208
- [60]. Epperly M.W., Sikora C., Defilippi S., Gretton J., Zhan Q., Kufe D.W., et al: MnSOD inhibits irradiation-induced apoptosis by stabilization of the mitochondrial membrane against the effects of SAP kinases p38 and Jnk1 translocation. Radiat Res 2002; 157: pp. 568-577
- [61]. Epperly M.W., Bernarding M., Gretton J., Jefferson M., Nie S., and Greenberger J.S.: Overexpression of the transgene for manganese superoxide dismutase (MnSOD) in 32D cl 3 cells prevents apoptosis induction by TNF-α, IL-3 withdrawal and ionizing irradiation. Exp Hematol 2003; 31: pp. 465-474
- [62]. Epperly M.W., Sikora C.A., Defilippi S., Gretton J.E., and Greenberger J.S.: Bone marrow origin of myofibroblasts in irradiation pulmonary fibrosis. Am J Resp Mol Cell Biol 2003; 29: pp. 213-224
- [63]. Epperly M.W., Gretton J.E., Bernarding M., Nie S., Rasul B., and Greenberger J.S.: Mitochondrial localization of copper/zinc superoxide dismutase (Cu/ZnSOD) confers radioprotective functions . Radiat Res 2003; 160: pp. 568-578
- [64]. Kanai A., Epperly M.W., Pearce L., Birder L., Zeidel M., Meyers S., et al: Differing roles of mitochondrial nitric oxide synthase in cardiomyocytes and urothelial cells. Am J Physiol Heart Circ Physiol 2004; 286: pp. H13-H21
- [65]. Epperly M.W., Osipov A.N., Martin I., Kawai K., Borisenko G.G., Jefferson M., et al: Ascorbate as a “redox-sensor” and protector against irradiation-induced oxidative stress in 32D cl 3 hematopoietic cells and subclones overexpressing human manganese superoxide dismutase. Int J Radiat Oncol Biol Phys 2004; 58: pp. 851-861
- [66]. Tyurin V.A., Tyurina Y.Y., Jung M.-Y., Tungekar M.A., Wasserloos K.J., Bayir H., et al: Mass-spectrometric analysis of hydroperoxy- and hydroxy-derivatives of cardiolipin and phosphatidylserine in cells and tissues induced by proapoptotic and pro-inflammatory stimuli. J Chromatogr B 2009; 877: pp. 2863-2879
- [67]. Kagan V.E., Wipf P., Stoyanovsky D., Greenberger J.S., Borisenko G., Belikova N.A., et al: Mitochondrial targeting of electron scavenging antioxidants: regulation of selective oxidation vs random chain reactions. Adv Drug Deliv Rev 2009; 61: pp. 1375-1385
- [68]. Stoyanovsky D.A., Vlasova I.I., Belikova N.A., Kapralov A., Tyurin V., et al: Targeting and activation of NO donors in mitochondria: peroxidase metabolism of (2-hydroxyamino-vinyl)-triphenyl-phosphonium by cytochrome c releases NO and protects cells from apoptosis. FEBJ Lett 2009; 583: pp. 2000-2005
- [69]. Belikova N.A., Glumac A., Rafikov R., Jiang J., Greenberger J.S., Kagan V.E., et al: Radioprotection by short-term oxidative preconditioning: role of manganese superoxide dismutase. FEBS Lett 2009; 583: pp. 3437-3442
- [70]. Bernard M.E., Kim H., Epperly M.W., Franicola D., Zhang X., Houghton F., et al: GS-nitroxide (JP4-039) mediated radioprotection of human Fanconi anemia cell lines. Radiat Res 2011; 176: pp. 603-612
- [71]. Helleday T., Lo J., van Gent D.C., and Engelward B.P.: DNA double-strand break repair: from mechanistic understanding to cancer treatment. DNA Repair (Amst) 2007; 6: pp. 923-935
- [72]. Kagan V.E., Tyurina Y.Y., Bayir H., Chu C.T., Kapralov A.A., Vlasova I.I., et al: The “pro-apoptotic genies” get out of mitochondria: oxidative lipidomics and redox activity of cytochrome c/cardiolipin complexes. Chem Biol Interact 2006; 163: pp. 15-28
- [73]. Belikova N.A., Jiang J., Tyurina Y.Y., Zhao Q., Epperly M.W., Greenberger J., et al: Cardiolipin specific peroxidase reactions of cytochrome c in mitochondria during irradiation induced apoptosis. Int J Radiat Oncol Biol Phys 2007; 69: pp. 176-185
- [74]. Kagan V.E., Bayir H.A., Belikova N.A., Kapralov O., Tyurina Y.Y., Tyurin V.A., et al: Cytochrome c/cardiolipin relations in mitochondria: a kiss of death. Free Radic Biol Med 2009; 46: pp. 1439-1453
- [75]. Rwigema J.-C., Beck B., Wang W., Doemling A., Epperly M.W., Shields D., et al: Two strategies for the development of mitochondrial-targeted small molecule radiation damage mitigators. Int J Radiat Oncol Biol Phys 2011; 80: pp. 860-868
- [76]. Bayir H., Fadeel B., Palladino M.J., Witasp E., Kurnikov I.V., Tyurina Y.Y., et al: Apoptotic interactions of cytochrome c: redox flirting with anionic phospholipids within and outside of mitochondria. Biochim Biophys Acta 2006; 1757: pp. 648-659
- [77]. Jiang J., Kurnikov I., Belikova N.A., Xiao J., Zhao Q., Vlasova I.L., et al: Structural requirements for optimized delivery, inhibition of oxidative stress and anti-apoptotic activity of targeted nitroxides. J Pharmacol Exp Ther 2007; 320: pp. 1050-1060
- [78]. Kim H., Bernard M.E., Epperly M.W., Shen H., Amoscato A., Dixon T.M., et al: Amelioration of radiation esophagitis by orally administered p53/mdm2/mdm4 inhibitor (BEB55) or GS-nitroxide. In Vivo 2011; 25: pp. 841-848
- [79]. Tyurina Y.Y., Tyurin V.A., Kapralova V.I., Wasserloos K., Mosher M., Epperly M., et al: Oxidative lipidomics of γ-irradiation induced lung injury: mass-spectrometric characterization of cardiolipin and phosphatidylserine peroxidation. Radiat Res 2011; 175: pp. 610-621
- [80]. Tyurina Y.Y., Tyurin V.A., Epperly M.W., Greenberger J.S., and Kagan V.E.: Oxidative lipidomics of γ-irradiation induced intestinal injury. Free Radic Biol Med 2008; 44: pp. 299-314
- [81]. Tyurin V.A., Tyurina Y.Y., Kochanek P.M., Hamilton R., DeKosky S.T., Greenberger J.S., et al: Oxidative lipidomics of programmed cell death. Methods Enzymol 2008; 442: pp. 375-393
- [82]. Tyurin V.A., Tyurina Y.Y., Ritov V.B., Lysytsya A., Amoscato A.A., Kochanek P.M., et al: Oxidative lipidomics of apoptosis: quantitative assessment of phospholipid hydroperoxides in cells and tissues. Mol Biol 2010; 610: pp. 353-374
- [83]. Rajagopalan M.S., Gupta K., Epperly M.W., Franicola D., Zhang X., Wang H., et al: The mitochondria-targeted nitroxide JP4-039 augments potentially lethal irradiation damage repair. In Vivo 2009; 23: pp. 717-726
- [84]. Belikova N.A., Jiang J., Stoyanovsky D.A., Greenberger J.S., and Kagan V.E.: Mitochondria-targeted (2-hydroxyamino-vinyl)-triphenyl-phosphonium releases NO and protects mouse embryonic cells against irradiation-induced apoptosis. FEBS Lett 2009; 583: pp. 1945-1950
- [85]. Epperly M.W., Rwigema J.C., Li S., Gao X., Wipf P., Goff , et al: Intraesophageal administration of GS-nitroxide (JP4-039) protects against ionizing irradiation-induced esophagitis. In Vivo 2010; 24: pp. 811-821
- [86]. Goff J.P., Epperly M.W., Shields D., Wipf P., Dixon T., and Greenberger J.S.: Radiobiologic effects of GS-nitroxide (JP4-039) in the hematopoietic syndrome. In Vivo 2011; 25: pp. 315-324
- [87]. Rubin P., Johnston C.J., Williams J.P., McDonald S., and Finkelstein J.N.: A perpetual cascade of cytokines postirradiation leads to pulmonary fibrosis. Int J Radiat Oncol Biol Phys 1995; 33: pp. 99-109
- [88]. Garipagaoglu M., Munley M.T., Hollis D., Poulson J.M., Bentel G.C., Sibley G., et al: The effect of patient-specific factors on radiation-induced regional lung injury. Int J Radiat Oncol Biol Phys 1999; 45: pp. 331-338
- [89]. Anscher M.S., King F.M., Andrews K., Clough R., Marks L.B., Bentel G., et al: Plasma TFGβ1 as a predictor of radiation pneumonitis. Int J Radiat Oncol Biol Phys 1998; 41: pp. 1029-1035
- [90]. Epperly M.W., Travis E.L., Sikora C., and Greenberger J.S.: Magnesium superoxide dismutase (MnSOD) plasmid/liposome pulmonary radioprotective gene therapy: modulation of irradiation-induced mRNA for IL-1, TNF-α, and TGF-β correlates with delay of organizing alveolitis/fibrosis. Biol Blood Marrow Transplant 1999; 5: pp. 204-214
- [91]. Epperly M.W., Zhang X., Nie S., Cao S., Kagan V., Tyurin V., et al: MnSOD–plasmid liposome gene therapy effects on ionizing irradiation induced lipid peroxidation of the esophagus. In Vivo 2005; 19: pp. 997-1004
- [92]. Epperly M.W., Franicola D., Zhang X., Nie S., Wang H., Bahnson A., et al: Decreased irradiation pulmonary fibrosis in Smad3 . In Vivo 2006; 20: pp. 573-582
- [93]. Epperly M.W., Shields D., Franicola D., Zhang X., Cao S., Carlos T., et al: Bone marrow from CD18 . In Vivo 2006; 20: pp. 431-438
- [94]. Zhang X., Epperly M.W., Kay M.A., Chen Z.-Y., Smith T., Franicola D., et al: Radioprotection . Hum Gene Ther 2008; 19: pp. 820-826
- [95]. Niu Y., Wang H., Wiktor-Brown D., Rugo R., Shen H., Huq M.S., et al: Irradiated esophageal cells are protected from radiation-induced recombination by MnSOD gene therapy. Rad Res 2010; 173: pp. 453-461
- [96]. Rajagopalan Malolan S., Stone B., Rwigema J.-C., Salimi U., Epperly M.W., Goff J., et al: Intraesophageal manganese superoxide dismutase-plasmid liposomes ameliorates novel total body and thoracic irradiation sensitivity of homologous deletion recombinant negative nitric oxide synthase-1 (NOS1 . Radiat Res 2010; 174: pp. 297-312
- [97]. Pearce L.L., Epperly M.W., Greenberger J.S., Pitt B., and Peterson J.: Identification of respiratory complexes I and III as mitochondrial sites of damage following exposure to ionizing radiation and nitric oxide. Nitric Oxide 2001; 5: pp. 128-136
- [98]. Greenberger J.S., and Epperly M.W.: Antioxidant therapeutic approaches toward amelioration of the pulmonary pathophysiological damaging effects of ionizing irradiation. Curr Respir Med Rev 2007; 3: pp. 29-37
- [99]. Zwacka R.M., Dudus L., Epperly M.W., Greenberger J.S., and Engelhardt J.F.: Redox gene therapy protects human IB-3 lung epithelial cells against ionizing radiation-induced apoptosis. Hum Gene Ther 1998; 9: pp. 1381-1386
- [100]. Epperly M.W., Bray J.A., Krager S., Berry L.A., Gooding W., Engelhardt J.F., et al: Intratracheal injection of adenovirus containing the human MnSOD transgene protects athymic nude mice from irradiation-induced organizing alveolitis. Int J Radiat Oncol Phys 1999; 43: pp. 169-181
- [101]. Epperly M.W., Bray J.A., Esocobar P., Bigbee W.L., Watkins S., and Greenberger J.S.: Overexpression of the human MnSOD transgene in subclones of murine hematopoietic progenitor cell line 32D cl 3 decreases irradiation-induced apoptosis but does not alter G . Radiat Oncol Invest Clin Basic Res 1999; 7: pp. 331-342
- [102]. Stickle R.L., Epperly M.W., Klein E., Bray J.A., and Greenberger J.S.: Prevention of irradiation-induced esophagitis by plasmid/liposome delivery of the human manganese superoxide dismutase (MnSOD) transgene. Radiat Oncol Invest Clin Basic Res 1999; 7: pp. 204-217
- [103]. Epperly M.W., Sikora C., Defilippi S., Bray J., Koe G., Liggitt D., et al: Plasmid/liposome transfer of the human manganese superoxide dismutase (MnSOD) transgene prevents ionizing irradiation-induced apoptosis in human esophagus organ explant culture. Int J Cancer 2000; 90: pp. 128-137
- [104]. Epperly M.W., Defilippi S., Sikora C., Gretton J., Kalend K., and Greenberger J.S.: Intratracheal injection of manganese superoxide dismutase (MnSOD) plasmid/liposomes protects normal lung but not orthotopic tumors from irradiation. Gene Ther 2000; 7: pp. 1011-1018
- [105]. Epperly M.W., Epstein C.J., Travis E.L., and Greenberger J.S.: Decreased pulmonary radiation resistance of manganese superoxide dismutase (MnSOD)-deficient mice is corrected by human manganese superoxide dismutase-plasmid/liposome (SOD2-PL) intratracheal gene therapy. Radiation Res 2000; 154: pp. 365-374
- [106]. Epperly M.W., Gretton J.A., DeFilippi S.J., Sikora C.A., Liggitt D., Koe G., et al: Modulation of radiation-induced cytokine elevation associated with esophagitis and esophageal stricture by manganese superoxide dismutase-plasmid/liposome (SOD-PL) gene therapy. Radiat Res 2001; 155: pp. 2-14
- [107]. Epperly M.W., Travis E.L., Whitsett J.A., Epstein C.J., and Greenberger J.S.: Overexpression of manganese superoxide dismutase (MnSOD) in whole lung or alveolar type II (AT-II) cells of MnSOD transgenic mice does not provide intrinsic lung irradiation protection. Radiat Oncol Invest 2001; 96: pp. 11-21
- [108]. Greenberger J.S., Kagan V.E., Pearce L., Boriseniao G., Tyurina Y., and Epperly M.W.: Modulation of redox signal transduction pathways in the treatment of cancer. Antioxid Redox Signal 2001; 3: pp. 347-359
- [109]. Li S., Yan T., Yan J.-Q., Oberley T.D., and Oberley L.W.: The role of cellular glutathione peroxidase redox regulation in the suppression of tumor cell growth by manganese superoxide dismutase. Cancer Res 2000; 60: pp. 3927-3939
- [110]. Epperly M.W., Sikora C.A., DeFilippi S.J., Gretton J.E., Bar-Sagi D., Carlos T., et al: Pulmonary irradiation-induced expression of VCAM-1 and ICAM-1 is decreased by MnSOD-PL gene therapy. Biol Blood Bone Marrow Transplant 2002; 8: pp. 175-187
- [111]. Epperly M.W., Guo H.L., Jefferson M., Wong S., Gretton J., Bernarding M., et al: Cell phenotype specific duration of expression of epitope-tagged HA-MnSOD in cells of the murine lung following intratracheal plasmid liposome gene therapy. Gen Ther 2003; 10: pp. 163-171
- [112]. Epperly M.W., Guo H.L., Bernarding M., Gretton J., Jefferson M., and Greenberger J.S.: Delayed intratracheal injection of manganese superoxide dismutase (MnSOD)-plasmid/liposomes provides suboptimal protection against irradiation-induced pulmonary injury compared to treatment before irradiation. Gene Ther Mol Biol 2003; 7: pp. 61-68
- [113]. Epperly M.W., Hongliang G., Shields D., Zhang X., Flanders K., Lambert P., et al: Correlation of ionizing irradiation-induced late pulmonary fibrosis with long-term bone marrow culture fibroblast progenitor cell biology in mice homozygous deletion recombinant negative for endothelial cell adhesion molecules. In Vivo 2004; 18: pp. 1-14
- [114]. Bernard M.E., Kim H., Rajagopalan M.S., Stone B., Salimi U., Rwigema J.-C., et al: Repopulation of the irradiation damaged lung with marrow derived cells. In Vivo 2012; 26: pp. 9-18
- [115]. Guo H., Epperly M.W., Bernarding M., Nie S., Gretton J., Jefferson M., et al: Manganese superoxide dismutase-plasmid/liposome (MnSOD-PL) intratracheal gene therapy reduction of irradiation-induced inflammatory cytokines does not protect orthotopic Lewis lung carcinomas. In Vivo 2003; 17: pp. 13-22
- [116]. Epperly M.W., Wegner R., Kanai A.J., Kagan V., Greenberger E.E., Nie S., et al: Irradiated murine oral cavity orthotopic tumor antioxidant pool destabilization by MnSOD-plasmid liposome gene therapy mediates tumor radiosensitization. Radiat Res 2007; 267: pp. 289-297
- [117]. St. Clair D.K., Oberley T.D., Muse K.E., and St. Clair W.H.: Expression of manganese superoxide dismutase promotes cellular differentiation. Free Radic Biol Med 1994; 16: pp. 275-282
- [118]. Carpenter M., Epperly M.W., Agarwal A., Nie S., Hricisak L., Niu Y., et al: Inhalation delivery of manganese superoxide dismutase-plasmid/liposomes (MnSOD-PL) protects the murine lung from irradiation damage. Gene Ther 2005; 12: pp. 685-690
- [119]. Epperly M.W., Kagan V.E., Sikora C.A., Gretton J.E., Defilippi S.J., Bar-Sagi D., et al: Manganese superoxide dismutase-plasmid/liposome (MnSOD-PL) administration protects mice from esophagitis associated with fractionated irradiation. Int J Cancer 2001; 96: pp. 221-233
- [120]. Epperly M.W., Defilippi S., Sikora C., Gretton J., and Greenberger J.S.: Radioprotection of lung and esophagus by overexpression of the human manganese superoxide dismutase transgene. Mil Med 2002; 167: pp. 071
- [121]. Epperly M.W., Goff J.P., Sikora C.A., Shields D.S., and Greenberger J.S.: Bone marrow origin of cells with capacity for homing and differentiation to esophageal squamous epithelium. Radiat Res 2004; 162: pp. 233-240
- [122]. Epperly M.W., Shen H., Jefferson M., and Greenberger J.S.: [object Object]. In Vivo 2004; 18: pp. 675-685
- [123]. Epperly M.W., Shen H., Zhang X., Nie S., Cao S., and Greenberger J.S.: Protection of esophageal stem cells from ionizing irradiation by MnSOD-plasmid liposome gene therapy. In Vivo 2005; 19: pp. 965-974
- [124]. Niu Y., Epperly M.W., Shen H., Smith T., Lewis D., Gollin S., et al: Intraesophageal MnSOD-plasmid liposome administration enhances engraftment and self-renewal capacity of bone marrow derived progenitors of esophageal squamous epithelium. Gene Ther 2008; 15: pp. 347-356
- [125]. Guo H.L., Seixas-Silva J.A., Epperly M.W., Gretton J.E., Shin D.M., and Greenberger J.S.: Prevention of irradiation-induced oral cavity mucositis by plasmid/liposome delivery of the human manganese superoxide dismutase (MnSOD) transgene. Radiation Research 2003; 159: pp. 361-370
- [126]. Epperly M.W., Carpenter M., Agarwal A., Mitra P., Nie S., and Greenberger J.S.: Intra-oral manganese superoxide dismutase plasmid liposome radioprotective gene therapy decreases ionizing irradiation-induced murine mucosal cell cycling and apoptosis. In Vivo 2004; 18: pp. 401-410
- [127]. Kanai A.J., Zeidel M.L., Lavelle J.P., Greenberger J.S., Birder L.A., de Groat W.C., et al: Manganese superoxide dismutase gene therapy protects against irradiation-induced cystitis. Am J Physiol 2002; 44: pp. 1152-1160
- [128]. Guo H.L., Wolfe D., Epperly M.W., Huang S., Liu K., Glorioso J.C., et al: Gene transfer of human manganese superoxide dismutase protects small intestinal villi from radiation injury. J Gastrointest Surg 2003; 7: pp. 229-236
- [129]. Epperly M.W., Smith T., Zhang X., Greenberger B., Komanduri P., Wang H., et al: Modulation of . Gene Ther 2010; 18: pp. 579-583
- [130]. Gokhale A.S., Epperly M., Glowacki J., Wang H., Wipf P., Pierce J.G., et al: Small molecule GS-nitroxide and MnSOD gene therapy ameliorate ionizing irradiation-induced delay in bone wound healing in a novel murine model. In Vivo 2010; 24: pp. 377-386
- [131]. Greenberger J.S.: Gene therapy approaches for stem cell protection. Gene Ther 2008; 15: pp. 100-108
- [132]. Epperly M.W., Epperly L.D., Zhang X., Franicola D., and Greenberger J.S.: Overexpression of MnSOD transgene product protects cryopreserved bone marrow hematopoietic progenitor cells from ionizing irradiation. Radiat Res 2007; 168: pp. 560-566
- [133]. Epperly M.W., Smith T., Wang H., Schlesselman J., Franicola D., and Greenberger J.S.: Modulation of total body irradiation induced life shortening by systemic intravenous MnSOD-plasmid liposome gene therapy. Radiat Res 2008; 170: pp. 437-444
- [134]. Greenberger J.S., and Epperly M.: Bone marrow-derived stem cells and radiation response. Semin Radiat Oncol 2009; 19: pp. 133-139
- [135]. Epperly M.W., Wang H., Jones J., Dixon T., Montesinos C., and Greenberger J.S.: Antioxidant-chemoprevention diet ameliorates late effects of total body irradiation and supplements radioprotection by MnSOD-plasmid liposome administration. Radiat Res 2011; 175: pp. 759-765
- [136]. Epperly M.W., Lai S.M., Mason N., Lopresi B., Dixon T., Franicola D., et al: Effectiveness of combined modality radiotherapy of orthotopic human squamous cell carcinomas in Nu/Nu mice using cetuximab, tirapazamine, and MnSOD-plasmid liposome gene therapy. In Vivo 2010; 24: pp. 1-8
- [137]. Tarhini A.A., Belani C., Luketich J.D., Ramalingam S.S., Argiris A., Gooding W., et al: A phase I study of concurrent chemotherapy (paclitaxel and carboplatin) and thoracic radiotherapy with swallowed manganese superoxide dismutase (MnSOD) plasmid liposome (PL) protection in patients with locally advanced stage III non-small cell lung cancer. Hum Gene Ther 2011; 22: pp. 336-343
- [138]. Kim H., Epperly M.W., Flickinger J., Bernard M., and Greenberger J.S.: The pro-autophagy drug carbamazepine is a radiation protector and mitigator. Int J Rad Biol 2011; 87: pp. 1052-1060
- [139]. Fein D.E., Limberis M.P., Maloney S.F., Heath J.M., Wilson J.M., and Diamond S.L.: Cationic lipid formulations alter the . Mol Ther 2009; 17: pp. 2078-2087
- [140]. Sinn P.L., Burnight E.R., and McCray P.B.: Progress and prospects: prospects of repeated pulmonary administration of viral vectors. Gene Ther 2009; 16: pp. 1059-1065
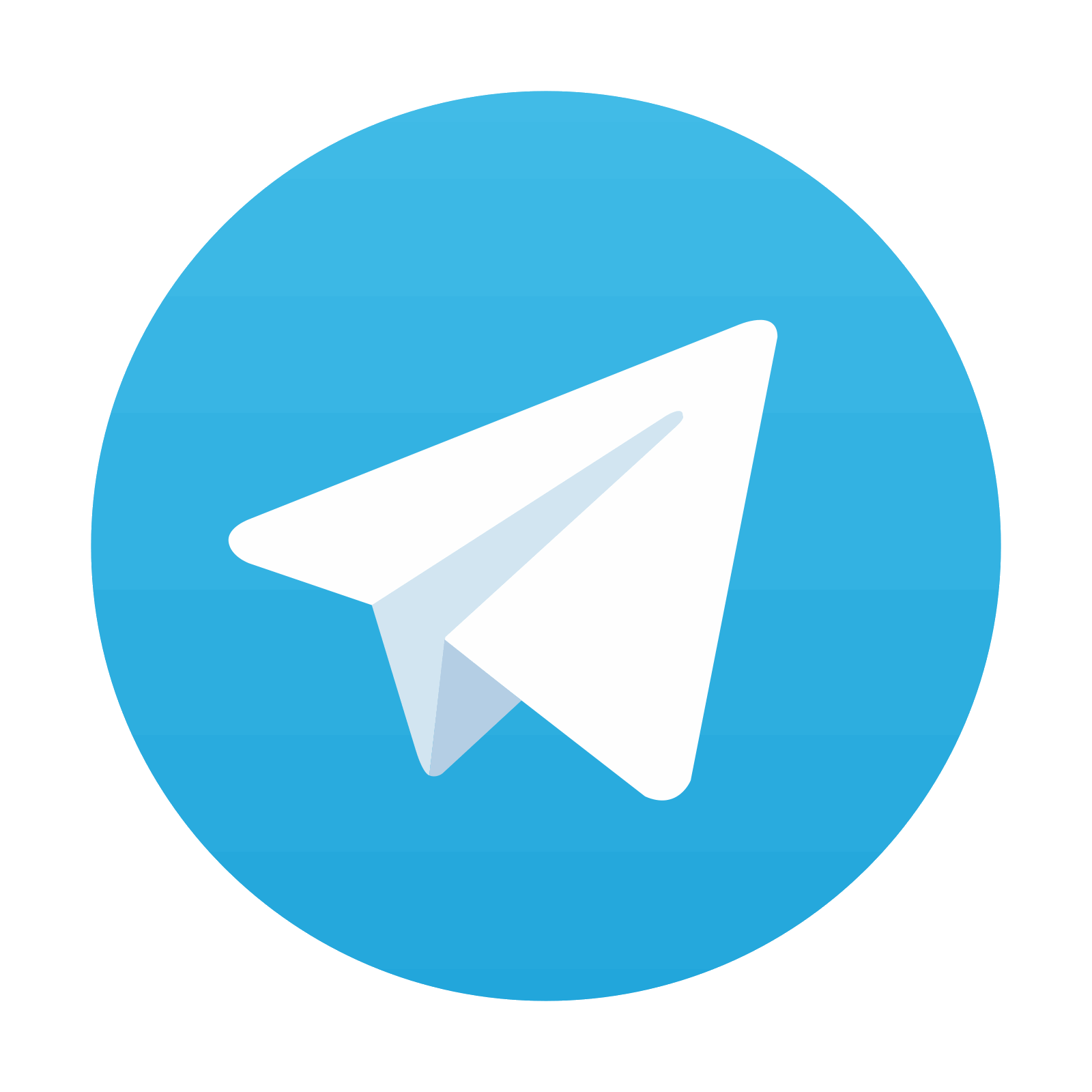
Stay updated, free articles. Join our Telegram channel
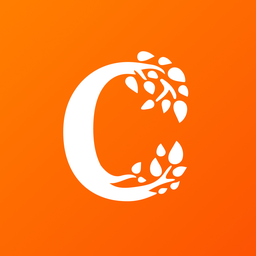
Full access? Get Clinical Tree
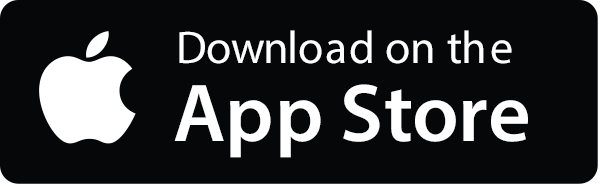
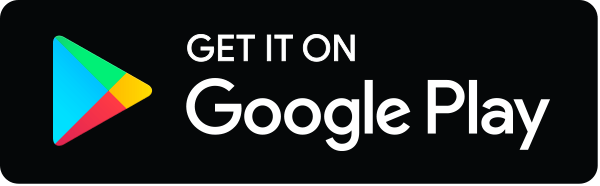