Function
Complication
Therapy-related risks
Relationship to time, dose to gland or organ when applicable
Linear Growth
GH deficiency
Surgery
Damage to the pituitary by tumor expansion and/or surgery
Radiotherapy to hypothalamus or pituitary
Doses > 30 Gy, effect by 5 years after exposure
Doses 18–24 Gy, effect may appear >10 years following exposure
Puberty
Central Precocious Puberty
Radiotherapy to hypothalamus or pituitary
Doses ≥18 Gy, Girls <5 years old at exposure have a higher risk
Hypogonadotropic Hypogonadism
Surgery
Damage to the pituitary by tumor expansion and/or surgery
Radiotherapy to hypothalamus or pituitary
Doses >30 Gy
Decreased fertility (partial deficit?) 22–27 Gy
Pituitary, other
ACTH deficiency
Surgery
Damage to the pituitary by tumor expansion and/or surgery
Radiotherapy to hypothalamus or pituitary
Doses >30 Gy
Systemic glucocorticoids
Deficiency depends on the doses used and duration of exposure
TSH deficiency
Surgery
Damage to the pituitary by tumor expansion and/or surgery
Radiotherapy to hypothalamus or pituitary
Doses >30 Gy
Thyroid
Primary hypothyroidism
Radiotherapy to the neck
Risk increases with dose (even <35 Gy) and time after exposure
I131 labeled agents
Such as MIBG for neuroblastoma
Hyperthyroidism
Radiotherapy to the neck
Doses >35 Gy
Auto-immune diseases
HSCT
Transfer of monoclonal antibodies from donor to recipient
Cancer
Radiotherapy to the neck
Doses 20–29 Gy; age <10 years at exposure, latency can be >20 years
Testes
Leydig cell function
Alkylating agents
Generally subclinical
Radiotherapy to testes
Doses >24 Gy
Germ cell function
Alkylating agents
Cyclophosphamide dose >7.5 g/m2
Radiotherapy to testes
Possible >0.15 Gy, high risk >2 Gy
Ovaries
Ovarian failure
Alkylating agents
Higher risk for older age at exposure
Radiotherapy to pelvis
Acute ovarian failure at doses >20 Gy. Premature menopause/infertility at lower doses; higher risk for older age at exposure.
Bone
Osteoporosis
Chemotherapy
Methotrexate, systemic glucocorticoids
Radiotherapy
TBI
Associated deficits
Untreated hypogonadism, GH deficiency, nutritional causes.
Metabolism
Obesity and insulin resistance
Surgery to hypothalamus
Large resection causes “central obesity”
Radiotherapy
Cranial radiotherapy, TBI.
Table 6.2
Elements of clinical suspicion and screening tools for common endocrine late effects
Condition | Clinical Suspicion | Laboratory Studies | Intervention |
---|---|---|---|
GH Deficiency | Risk exposure (surgery, cranial radiotherapy) | Bone Age | GH replacement |
Decreased growth velocity, growth failure. | IGF1, IGF-BP3 | ||
GH Stimulation Test | |||
Central Precocious Puberty | Tanner stage 2 before 8 y/o in girls | Bone Age | GnRH agonist |
Menarche before the age of 10 y/o | Leuprolide Stimulation Test | ||
Tanner stage 2 before 9 y/o in boys | |||
Hypogonadotropic Hypogonadism | Tanner stage 1 past 12 y/o in girls | Baseline LH, FSH | Induction of puberty/Sex hormone replacement |
Primary or secondary amenorrhea | Estradiol (girls) | ||
Tanner stage 1 past 13 y/o in boys | Testosterone (boys) | ||
Arrested Puberty | |||
Central Adrenal Deficiency | Risk exposure (surgery, high dose cranial radiotherapy, prolonged systemic steroids) | 8 AM cortisol and ACTH | Hydrocortisone and stress dose teaching. |
Presence of other pituitary deficits | Low dose ACTH stimulation test if AM cortisol abnormal. | ||
Clinical signs of adrenal insufficiency | |||
Hypothyroidism | Risk exposure (surgery, high dose cranial radiotherapy, radiotherapy to the neck, I131 labeled agents). | TSH, Free T4 | Levothyroxine |
Presence of other pituitary deficits | |||
Clinical signs of hypothyroidism | |||
Thyroid Neoplasms | Risk exposure (Radiotherapy to the neck) | Thyroid ultrasound (US) | Per etiology |
Nodule on careful palpation of neck. | US guided FNAB | ||
Leydig Cell Failure, males | Tanner stage 1 past 13 y/o | Baseline LH, FSH | Induction of puberty/Sex hormone replacement |
Arrested puberty | Testosterone | ||
Low androgen symptoms | |||
Germ Cell Failure, males | Decreased/no progression of testicular volume | Baseline FSH, inhibin B | Sperm banking pre-therapy |
Adults:Sperm count | |||
Ovarian Failure | Tanner stage 1 past 12 y/o | Baseline LH, FSH | Induction of puberty/Sex hormone replacement |
Arrested puberty | Estradiol | ||
Primary or secondary amenorrhea | Adults:AMH | ||
Bone Health | History of fractures | BMD studies vitamin D 25 levels | Per etiology |
Dietary restrictions | |||
Multiple hormonal deficiencies | |||
Obesity, Overweight | BMI > 85th percentile in children | Fasting glucose, lipids, insulin levels. | Diet, active lifestyle. |
BMI > 25 kg/m2 in adults | Hemoglobin A1c | ||
Evidence of abdominal obesity | Oral glucose tolerance test if fasting tests abnormal. |
6.2 Disorders of the Hypothalamus and Pituitary
6.2.1 Growth Hormone Deficiency
The potential for linear growth can be eroded by various endocrine and non-endocrine factors, leading to adult short stature. Growth hormone (GH) deficiency is among the main endocrine factors associated with short stature and most frequently occurs as a complication of cranial radiotherapy.
Tumors developing close to the hypothalamus and/or pituitary gland such as craniopharyngiomas, germinomas, and optic nerve gliomas can cause GH deficiency as a direct anatomical insult or because of the surgery required to remove or reduce the size of these tumors. The other hypothalamic–pituitary functions are generally affected in these situations.
Growth hormone deficiency is the most frequently observed and often the only hypothalamic–pituitary deficit in individuals treated with cranial radiotherapy [4, 5]. The deficit occurs in a time- and dose-dependent fashion. The higher the dose of radiation and the longer the time interval from treatment, the greater the risk [6]. Growth hormone deficiency can be observed within 5 years when doses exceed 30 Gy [5]. Following lower doses, such as 18 to 24 Gy, growth hormone deficiency may not become evident for 10 or more years [7]. The effects of chemotherapy alone on GH secretion are not as well established as those of radiotherapy. Growth hormone deficiency has been reported in relatively small numbers of individuals treated with chemotherapy alone [8–11].
The diagnosis of GH deficiency can be difficult to establish except in situations where direct anatomical insults to the hypothalamus and/or the pituitary are documented (e.g., sellar tumors, surgical removal). Given the kinetics of GH secretion, the hormone being released mostly through nocturnal pulses, deficiencies can be difficult to capture and the diagnosis has to be based on the convergence of clinical features and laboratory results. A decreased growth velocity, observed over a 6-month time interval, should raise the suspicion of GH deficiency [12]. It is important to note that other endocrine and non-endocrine factors can contribute to growth deceleration and that the elements obtained during the clinical evaluation can help in elucidating the contribution of each additional factor. For instance, high dose radiotherapy to the spine, such as following total body irradiation (TBI), can directly damage the vertebral growth plates and cause a skeletal dysplasia where the sitting height is more affected than the standing height [13–16]. It is therefore important in individuals who received such treatments to measure and plot on dedicated growth charts the sitting height of the individual and compare this to the standing height. Body weight and body mass index (BMI) are important markers of nutritional status which can influence linear growth. In addition, excessive weight gain contrasting with linear growth deceleration can reflect thyroid dysfunction. Pubertal staging is an important part of any endocrine evaluation, but is essential in this context as concurrent central precocious puberty—a fairly common endocrine complication observed in individuals treated with cranial radiotherapy—can mask the clinical signs of growth hormone deficiency with seemingly normal growth rates owing to the inappropriate secretion of sex steroids.
Given the pulsatile and circadian nature of GH secretion patterns, GH plasma measurements require dynamic testing, often referred to as GH stimulation testing. A pharmacologic agent known to increase GH secretion (or secretagogue) is injected and GH levels are measured serially over a 2-hour period. Stimulation tests are non-physiologic and can yield non-reproducible results. In children, the interpretation of the GH peak values varies with the GH assay and the standard used by the different laboratories; a normal GH peak response hence varies between 5 and 10 ng/mL depending on all these factors. In adults, different cut off values have been defined depending on the secretagogue used during the test and according to BMI [17]. The diagnosis of GH deficiency generally requires failing two stimulation tests using two different secretagogues. In patients who received irradiation to the hypothalamus and/or pituitary, however, failing one stimulation test was considered enough in the consensus guidelines published by the Growth Hormone Research Society [18]. Plasma Insulin like growth factor-1 (IGF-1) and IGF binding protein-3 (IGFBP-3) which are more stable than GH levels, are widely used as surrogate markers of GH secretion. They are used by many clinicians as screening tools before offering GH stimulation testing. This approach is problematic by several aspects. The interpretation of the IGF-1 and IGFBP-3 levels requires factoring in patient age, pubertal stage, and skeletal maturation data. Plasma IGF-1 levels are also affected by changes in liver function and by the nutritional status. Additionally, IGF-1 and IGFBP-3 levels can be inappropriately normal in children with GH deficiency following hypothalamic/pituitary irradiation or local tumoral expansion and hence cannot be used to screen for GH deficiency in this population [19, 20]. Hence, when clinical suspicion is present, GH stimulation testing should be offered even if IGF-1 and/or IGFBP-3 levels were within the normal range. A left-hand X-ray obtained to assess the degree of skeletal maturation, referred to as the “Bone Age” is an important part of the investigation of a child with growth deceleration. Differences between the bone age and the chronological age are helpful in assessing the potential for catch-up growth [21].
Replacement therapy using recombined human GH is proven to improve final height prospects in survivors with childhood onset GH deficiency [22, 23]. Initiation of replacement therapy at a younger bone age—and hence longer duration of replacement therapy—and higher doses of therapy positively correlated with a greater final height outcome [24]. Safety concerns pertaining to the use of GH in individuals with a history of malignancy are related to the known pro-mitogenic and proliferative effects of GH and IGF-1. Growth hormone replacement is generally deferred until 1 year after the successful completion of all cancer therapies and initiated only if there are no changes in the patient’s remission status. The treatment is also interrupted when a patient is diagnosed with a recurrence or a second neoplasm. In order to more systematically address these ongoing safety concerns, many large-scale studies pertaining to the long term risks associated with the use of GH in childhood cancer survivors have been published over the years. These studies did not show an increase in the risk of brain tumor recurrence, disease recurrence, or death following GH replacement therapy [25–27]. However, there was a slight increase in the risk of a secondary solid tumor, the most commonly observed being meningiomas [27, 28]. Cancer survivors treated with GH may also be at a higher risk of developing slipped epiphyses compared with children treated with GH for idiopathic GH deficiency [29]. As an entity, adult GH deficiency is increasingly being recognized given its association with increased body fat, raised plasma lipids, and decreased bone density and reduced quality of life [10, 11, 30]. Treatment with GH in adult survivors of childhood cancer seems to have a greater impact on quality of life, and to result in improvements in the metabolic parameters [11, 31–33].
6.2.2 Disorders of the Luteinizing Hormone (LH) and the Follicle Stimulating Hormone (FSH)
6.2.2.1 Central Precocious Puberty
Central precocious puberty (CPP) occurs following the premature activation of the hypothalamic–pituitary–gonadal axis [34]. Precocious puberty can lead to abnormally rapid skeletal maturation, with early fusion of the growth plates, hence contributing to the risk of adult short stature. In addition, a very early onset of puberty—of menstrual activity in girls in particular—can be a source of psychosocial stress leading to adjustment difficulties.
Cranial irradiation at both lower doses (18–35 Gy) and higher doses (>35 Gy) is associated with the development of central precocious puberty, by presumably disrupting inhibitory cortical influences [35–39]. In contrast, radiation doses >50 Gy are also associated with hypogonadotropic hypogonadism within the context of combined hormonal pituitary deficiencies [36, 39, 40]. Risk factors associated with CPP following hypothalamic irradiation include female sex, young age at treatment, and increased BMI [37, 41].
Precocious puberty is, first and foremost, a clinical diagnosis. It is defined by the onset of sustained pubertal development before the age of 8 years old in girls and before the age of 9 years old in boys [34]. The onset of pubertal development in girls is defined by the onset of breast development. In late referrals, the onset of menarche before the age of 10 years old is also considered an indicator of precocious puberty. In boys, the measurement of the testicular volume—used to assess the onset of pubertal development in the general population—may not be a reliable indicator of puberty in childhood cancer survivors, as chemotherapy and radiation can damage the seminiferous tubules, resulting in testes that are inappropriately small for a given stage of puberty. Thus, in boys, clinicians should be alerted by the early onset of other secondary sexual characters (e.g., pubic hair) prior to the age of 9 years and should not solely rely on testicular volume measurements. One of the first signs of pubertal development is an increase in the growth rate. In children who also are likely to have GH deficiency, this may result in falsely reassuring “normal” growth velocity.
Skeletal maturation can be assessed using the standard bone age (X-ray examination of the left wrist and hand) in order to estimate the individual’s skeletal age [21]. Advancement of the bone age more than two standard deviations (SD) for chronological age is a consistent finding in children with precocious puberty. In girls with CPP, uterine growth on the pelvic ultrasound is a sign of estrogen stimulation, and is an earlier finding than bilaterally enlarged ovaries. Gonadotropin secretion is best assessed using the gonadotropin releasing hormone (GnRH) or GnRH agonist stimulation tests. An ample luteinizing hormone (LH) response greater than the FSH response indicates a pubertal pattern. The plasma estradiol levels in girls and testosterone levels in boys are also important indicators of pubertal development. Various formulations are available for long acting GnRH agonists, such as the most commonly-used depot leuprolide acetate. These are used to effectively suppress LH and FSH secretion and stop the progression of puberty. This has been shown to improve the statural outcome, especially when contemporary regimens for GH replacement are used concurrently to treat GH deficiency [23].
6.2.2.2 Hypogonadotropic Hypogonadism
Hypogonadotropic hypogonadism is the clinical consequence of LH and FSH deficiencies. In individuals who have not completed pubertal development, hypogonadotropic hypogonadism results in delayed or arrested puberty. In individuals who had previously completed pubertal development it is among the causes of secondary amenorrhea in women and testosterone insufficiency in men.
Hypogonadotropic hypogonadism, as a complication of cranial radiotherapy, is less frequently observed than CPP. It tends to occur following the exposure of the hypothalamus/pituitary to high doses of radiation >30 to 40 Gy [4, 39, 42–44]. In female ALL survivors “subtle” defects of gonadotropin secretion following radiation doses in the 18–24 Gy range have been described [43, 45] while in a recent study, female survivors who received 22–27 Gy of hypothalamic/pituitary irradiation were found to have decreased fertility [46]; additional long-term follow-up data will provide a better sense of the ultimate effect of these lower doses.
In prepubertal individuals, hypogonadotropic hypogonadism can be clinically suspected through the observation of delayed puberty. This is defined by the absence of pubertal development (evidenced by breast development) past the age of 12 years old in girls. In boys, pubertal development should start prior to the age of 13 years old. As previously discussed, in boys, testicular volume may not be reliably used in the survivor population given the risk of a concomitant gonadal insult. Other markers may be useful, such as pubic hair or plasma testosterone levels. The clinical diagnosis in both sexes (and especially in males) can be difficult given the overlapping symptoms with constitutional delay of growth (simple pubertal delay). Mid-pubertal individuals would experience arrested puberty (no progression in Tanner staging and primary amenorrhea in girls). Post-pubertal women will experience secondary amenorrhea. Affected males will have symptoms of testosterone insufficiency such as reduced libido, erectile dysfunction, decreased bone mineral density (BMD), decreased muscle mass and other metabolic disturbances. The diagnosis can be further corroborated by low sex steroid levels associated with low LH and FSH levels. The treatment relies on the induction of pubertal development in prepubertal individuals and sex hormone replacement therapies in pubertal individuals.
6.2.3 Other Hypothalamic–Pituitary Deficiencies
6.2.3.1 Corticotropin Deficiency
Corticotropin (ACTH) deficiency in childhood cancer survivors is uncommon except when it occurs in the context of suppression by high dose systemic steroid medications. It can be observed along with other pituitary deficiencies as a result of local tumoral expansion or surgery or following high-dose (>30 Gy) radiation [5, 47, 48]. The symptoms evocative of central adrenal insufficiency include fatigue, nausea, vomiting, abdominal pain, hypotension, shock, unexplained clinical deterioration, vulnerability to infections and hypoglycemia. Laboratory investigations would reveal low plasma levels of cortisol and ACTH. Levels should be drawn as close to 8 AM as possible, although circadian variation is rarely present before the age of 3 months and may be impaired in severely ill patients. An 8 AM plasma cortisol <5 mcg/dL is suggestive of adrenal insufficiency while levels above 18 mcg/dL are sufficient to rule out the diagnosis [49]. Additional testing modalities include the insulin tolerance test, low dose ACTH and metyrapone stimulation tests. The treatment relies on replacement therapy with hydrocortisone and patient and family education in regards to glucocorticoid stress dosing.
6.2.3.2 Thyroid Stimulating Hormone (TSH) Deficiency
Thyroid stimulating hormone (TSH) deficiency, resulting in central hypothyroidism, occurs less often than GH deficiency and central precocious puberty following the irradiation of the hypothalamic/pituitary area. It has been reported following doses >30 to 40 Gy [4, 5, 50–52]. The clinical symptoms associated with hypothyroidism include fatigue, cold intolerance, abnormal weight gain, hair loss, low energy levels, depression, and menstrual irregularities. In children who have not completed growth, hypothyroidism is associated with a decreased growth velocity, contrasting with abnormal weight gain. Laboratory investigations are remarkable for a low Free T4 level with a TSH level that is either low or inappropriately within the normal range. The treatment is based on replacement with levothyroxine at substitutive doses. Free T4 levels are measured for dosage adjustment 6–8 weeks following the initiation of therapy and after any dosage adjustment; they can otherwise be followed every 6 months. Once the diagnosis is established and therapy is initiated, there is no need to monitor TSH levels in individuals with central hypothyroidism.
6.2.3.3 Hyperprolactinemia
Exposure of the hypothalamus to doses of radiation in the vicinity of 50 Gy and above can be associated with hyperprolactinemia [36]. Hyperprolactinemia is often mild and reported as a fortuitous laboratory finding. Hyperprolactinemia can suppress gonadotropin and cause hypogonadism in patients who otherwise have many reasons for gonadal dysfunction.
6.3 Disorders of the Thyroid
Thyroid disorders are common among childhood cancer survivors. These disorders include therapy-induced hypothyroidism and hyperthyroidism, thyroid autoimmune diseases and thyroid neoplasms.
6.3.1 Therapy Induced Primary Hypothyroidism
The thyroid gland is particularly vulnerable to radiation, and primary hypothyroidism is by far the most common thyroid disorder observed in childhood cancer survivors. Individuals treated with neck/mantle irradiation for Hodgkin lymphoma, craniospinal irradiation for brain tumors, or TBI for cytoreduction before HSCT are therefore at a significant risk of becoming hypothyroid in the years that follow the exposure [5, 7, 52–56]. Treatments with radio-labeled agents such as Iodine-131-metaiodobenzylguanidine (I131-MIBG) [57] and I131-labeled monoclonal antibody for neuroblastoma [58] have also been associated with hypothyroidism. Chemotherapy alone does not seem to cause hypothyroidism [56, 59]. The prevalence of hypothyroidism is primarily determined by the total dose of radiation to the thyroid and by the duration of follow-up. Additional risk factors for developing hypothyroidism include female gender, white race, and being older than 15 years of age at the time of diagnosis [54, 59]. As hypothyroidism can occur more than 25 years following the completion of cancer treatments, it is imperative that individuals at risk undergo lifelong surveillance.
Clinical signs suggestive of hypothyroidism include fatigue, cold intolerance, abnormal weight gain, hair loss, low energy levels, depression, and menstrual irregularities. In children who have not completed growth, hypothyroidism is associated with a decreased growth velocity contrasting with an abnormal weight gain. Given the relatively non-specific nature of many of these symptoms and knowing that many individuals would become profoundly hypothyroid before developing any of these symptoms, it is recommended to screen for abnormal thyroid functions in individuals at risk by obtaining labs at least yearly. These include plasma Free T4 and TSH levels. Elevated TSH levels contrasting with normal or low Free T4 levels are suggestive of primary hypothyroidism. The treatment consists of replacement with levothyroxine at substitutive doses.
6.3.2 Therapy Induced Hyperthyroidism
Therapy-induced hyperthyroidism is a relatively uncommon complication in childhood cancer survivors; it occurs most often following external beam radiation to the neck for Hodgkin lymphoma. The main risk factor is the exposure of the thyroid to doses >35 Gy [54, 56]. Medical treatment is determined individually depending on the severity of the presentation.
6.3.3 Autoimmune Thyroid Disease
Autoimmune thyroid disease, most likely a result of the adoptive transfer of abnormal clones of T or B cells from donor to recipient, has been reported in allogeneic HSCT recipients. Both hyperthyroidism and hypothyroidism have been described in the presence of TSH receptor auto-antibodies and thyroglobulin auto-antibody respectively [60, 61].
6.3.4 Thyroid Neoplasms
The exposure of the thyroid to either direct radiation or scatter radiation (for example after prophylactic CNS irradiation in patients treated for ALL) is a significant risk factor for thyroid neoplasms, benign and malignant. Treatment before 10 years of age and/or with doses in the range of 20–29 Gy are significant risk factors. The association between thyroid irradiation and thyroid neoplasms is linear at low doses of radiation, but shows a downward turn at doses above 20–25 Gy, with a risk that remains, nevertheless, elevated compared to the general population [62, 63]. The majority of the observed cancers were differentiated carcinoma (ie, papillary and follicular) with a median latency around 20 years [54, 62, 64]. An increased risk of thyroid cancer in association with chemotherapy (independent of radiotherapy) was recently reported but remains relatively minor compared to the effect of radiation [63].
In general, post-irradiation thyroid cancers behave in a non-aggressive fashion, similar to what is observed in de novo thyroid cancers among the young [65]. The pathogenesis of radiation-induced thyroid neoplasms is felt to be be related to rearrangements of RET-PTC induced by the exposure to radiation [66, 67]. Thyroid neoplasms following radiotherapy may not become evident for many years after exposure to radiation; therefore, all individuals at risk require lifelong clinical follow-up by an expert endocrinologist [54, 56, 65]. Periodically screening individuals at risk for the presence of clinically non-palpable thyroid neoplasms using thyroid ultrasound has been recommended by some groups [68]. This strategy remains controversial, as it may result in more frequent biopsies and has not, so far, been shown to reduce morbidity or mortality in this population [59].
6.4 Disorders of the Gonads
6.4.1 Males
The human testis harbors two functional compartments: A sex steroid-producing compartment, and a sperm-producing compartment. The former consists of testosterone-producing Leydig cells while the latter consists of the seminiferous tubules, which include germ cells as well as the Sertoli cells that support and nurture them. The two compartments are interrelated given that adequate testosterone production is necessary for normal spermatogenesis, but their functions otherwise remain relatively distinct [69]. The two functional compartments are affected in different ways by cancer treatments.
6.4.1.1 Leydig Cell Dysfunction
Leydig cell failure and testosterone insufficiency do not occur as frequently as germ dysfunction and infertility in childhood cancer survivors. Chemotherapy-induced Leydig cell failure requiring testosterone replacement therapy is rare [70, 71]. The deficit may be observed following treatment with alkylating agent regimens with some reports indicating that from 10 to 57 % of male subjects can develop elevated serum concentrations of LH following treatment [42, 71–78]. When it does occur, chemotherapy-induced Leydig cell dysfunction is generally subclinical and does not reach levels that require substitution with testoterone [9, 79, 80].
By contrast, Leydig cells are more vulnerable to radiation-induced damage. The interpretation of the specific impact of radiation on Leydig cell function is confounded by the concurrent use of chemotherapy and by the potential effects of the malignancy itself. Leydig cell failure, nevertheless, occurs at doses of radiation higher than those associated with germ cell dysfunction. The likelihood of sustaining radiation-induced Leydig cell failure is directly related to the dose delivered and inversely related to age at treatment [81–83]. Normal amounts of testosterone are produced by the majority of males who receive <20 Gy fractionated radiation to the testes [71]. A dose of >24 Gy of fractionated irradiation as therapy for young males with testicular relapse of ALL is associated with a very high risk for Leydig cell dysfunction. The majority of boys who are prepubertal at the time they receive 24 Gy testicular irradiation will develop Leydig cell failure and require androgen replacement [81]. Testicular doses in excess of 33 Gy have been associated with Leydig cell failure in 50 % of adolescent and young adult men [84].
In pre-pubertal boys, Leydig cell failure will result in delayed/arrested puberty and lack of secondary sexual characteristics. In sexually mature men, it can result in reduced libido, erectile dysfunction, decreased BMD, decreased muscle mass and other metabolic disturbances [71]. Raised plasma concentrations of LH combined with low levels of testosterone are characteristic of Leydig cell dysfunction. Given that LH and testosterone levels are generally undetectable or very low before the onset of puberty, it can be very difficult to assess or predict Leydig cell function in the preadolescent male. Therefore, these laboratory markers cannot be used until the individual has reached mid-adolescence. The treatment relies on replacement therapy with testosterone.
6.4.1.2 Germ Cell Dysfunction
With their high turnover rates, germ cells are more vulnerable to cancer treatments than Leydig cells; sperm production is frequently impaired by radiotherapy and several types of chemotherapy. Germ cell dysfunction with resultant infertility is often associated with reduced testicular volume, increased FSH concentrations, and reduced plasma concentrations of inhibin B [85, 86]. However, for clinical purposes and counseling, assessing male fertility requires obtaining a sperm count as none of these aforementioned surrogate markers has sufficient specificity or sensitivity to predict outcome for an individual subject [77, 87].
The chemotherapeutic agents most commonly associated with impaired male fertility are alkylating agents. Impaired fertility occurs in 40–60 % of young adult survivors of childhood cancer [77]. A high probability of oligospermia, azoospermia and infertility is associated with doses of cyclophosphamide >20 g/m2. In contrast, many individuals treated with a cumulative dose of 7.5–10 g/m2 or less retain normal sperm production [42, 77]. Procarbazine has also been shown to induce impaired sperm production in a dose-dependent fashion. Patients with Hodgkin lymphoma who received three mechlorethamine, vincristine, procarbazine, and prednisone (MOPP) cycles alternating with three cycles of doxorubicin hydrochloride, bleomycin, vinblastine, and dacarbazine seemed to suffer less testicular damage than patients who received 6 MOPP cycles [74, 88]. Most of the young men treated with the combination of busulfan and cyclophosphamide in preparation for HSCT do appear to sustain damage to their germinal epithelium, with possible recovery for patients treated at lower doses (120 mg/kg for cyclophosphamide and 16 mg/kg for busulfan) [89, 90].
Impaired sperm production can occur at doses of radiation as low as 0.15 Gy. If the dose is under 1–2 Gy, recovery is common. At doses >2 to 3 Gy, recovery of sperm production is rare [91]. Germ cell dysfunction is present in essentially all males treated with TBI [92]. Given the high rate of impaired sperm production in survivors, sperm banking should be offered to all adolescent males prior to the initiation of cancer therapy, whenever clinically feasible.
6.4.2 Females
In females, the estrogen producing cells and oocytes are functionally and structurally interdependent within the ovarian follicle. As a result, when ovarian failure occurs, both sex hormone production and fertility are disrupted [71]. Given the progressive decline in oocyte reserve with increasing age, older age is an important risk factor for ovarian failure following childhood cancer and its treatments [71]. Owing to a greater follicular reserve, the ovaries of prepubertal girls are more resistant to chemotherapy-induced damage when compared to the ovaries of adults [89, 93, 94]. Nevertheless, certain chemotherapeutic agents, especially alkylating agents, given at high doses can cause ovarian failure, even in younger subjects [94–97]. Older age at treatment, exposure to procarbazine at any age, and exposure to cyclophosphamide between the ages of 13–20 years old were independent risk factors for acute ovarian failure (AOF) [94]. Females who receive high-dose myeloablative therapy with alkylating agents such as busulfan, melphalan, and thiotepa in preparation for HSCT are at high risk of developing ovarian failure [98]. The majority of prepubertal girls and adolescents receiving standard chemotherapy will fortunately maintain or recover ovarian function during the immediate post-treatment period [71, 99, 100]. In women who do retain or recover function following treatment with standard doses of alkylating agents, a subset will experience premature menopause when they reach their twenties and thirties [71, 76, 95, 101, 102]. Female survivors with a history of exposure to high doses of alkylating agents, to lomustine or cyclophosphamide, were less likely to experience a pregnancy when compared to sibling controls [44]. When female childhood cancer survivors treated with chemotherapy do get pregnant, no adverse pregnancy outcomes were identified [103].
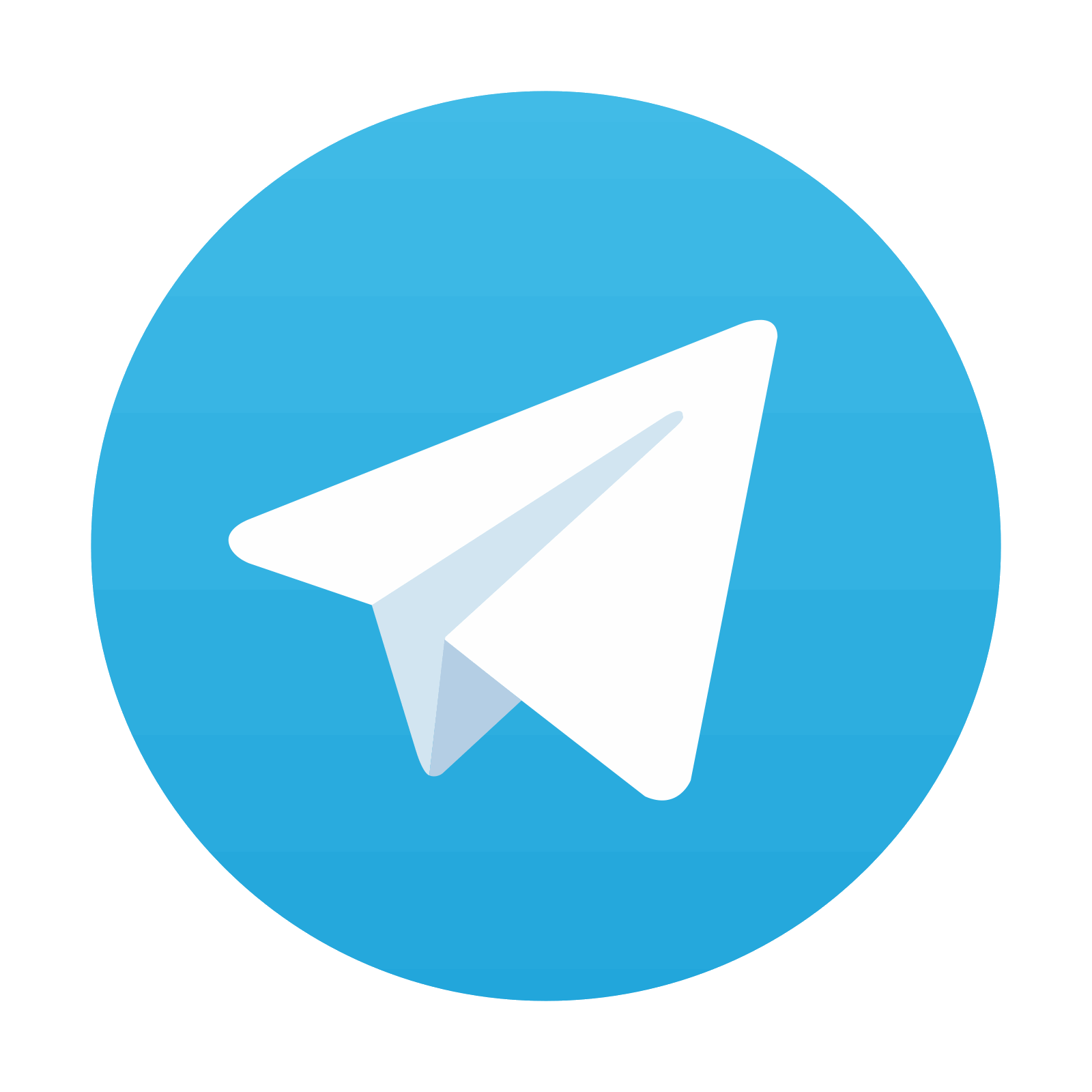
Stay updated, free articles. Join our Telegram channel
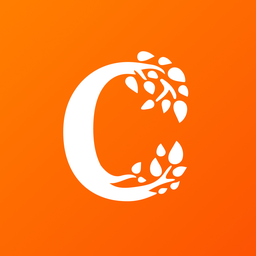
Full access? Get Clinical Tree
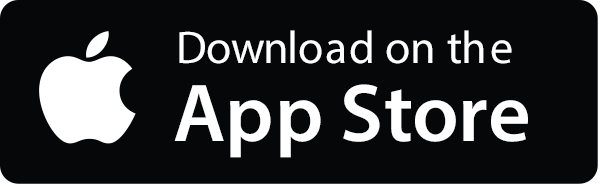
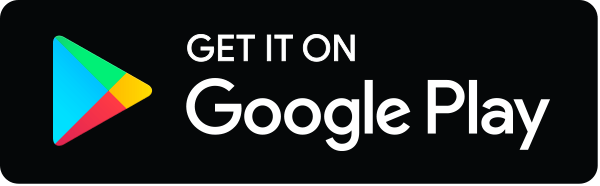