Keywords
Electroporation, gene therapy, cancer, nucleic acids, vaccine
Introduction
Electroporation or electropermeabilization (EP) is a physical method of introducing polar molecules such as DNA into eukaryotic cells through the cell membrane by exposing cells to electric pulses. The technique was first described by Neumann et al . in 1982 and is based on the earlier observation that biomembranes can be made transiently permeable when short electric impulses are applied . Since then, EP has been used to transport a variety of molecules, such as ions, drugs, dyes and tracers, and nucleic acids, across the cell membrane. During approximately the past 30 years, we have come a long way from transporting DNA across the plasma membrane in a simple in vitro setting. Electroporation can now be utilized in vivo for gene transfer, gene therapy, and chemotherapy. Since the late 1980s, a number of in vivo preclinical studies led to the first clinical study that used electroporation to deliver a chemotherapy drug, reported in 1993 . Since then, a number of other studies have used EP for electrochemotherapy and gene electrotransfer. The technology has been tested and successfully used in many clinical trials. Electrochemotherapy has been approved for clinical use in several European Union countries and is currently being practiced in more than 100 hospitals throughout Europe. In 2008, a book of protocols to support the use of electroporation in experimental and clinical gene medicine was published . This chapter explores the evolution of this technology from the early experiments to its current use in the clinic.
The Theory of Electroporation
Direct evidence for the exact mechanism of electroporation has yet to be elucidated, but based on in vitro experiments, the following explanation has been formulated. The contents of an animal cell are highly conductive and encased in a plasma membrane that is nonconductive. The membrane essentially insulates the inside of the cell from its surrounding environment, which is usually highly conductive. Applying an electric field that is greater than the membrane capacitance will cause charges of opposite polarity to line up on either side of the cell membrane and form a transmembrane potential difference at a specific point on the cell surface. Structural changes will be induced that result in the breakdown of the membrane that will allow molecules to pass. This renders the cell permeable to molecules to which it was previously impermeable. It is proposed that during this breakdown, water enters the membrane and transient hydrophilic areas are formed . If the applied field strength and pulse duration allow the defects to reseal, the cell remains viable. This is termed reversible electroporation . In vivo electroporation is largely thought of as reversible. If, however, the electroporation parameters are such that the defects are not allowed to reseal and cell death results, this is termed irreversible electroporation . Both preclinical and clinical in vivo studies have utilized irreversible electroporation to destroy cancer cells . This technique is a useful tool for cancer therapy because it induces targeted cell necrosis and can be used as an alternative to currently available toxic therapies.
When attempting to achieve permeabilization, one should take into consideration the size of the cell and its polar angle with respect to the electric field. The smaller the cell, the larger the external field needed for membrane permeabilization. The extent and degree of permeabilization can be controlled by the amplitude and the duration of the pulse, respectively . The greater the amplitude and duration of the pulse, the greater the degree of disruption of the membranes in the affected area. Ultrashort or nanosecond pulses delivered at high voltages result in the formation of nanopores in the cell membrane . A recent study reported that it may be possible to deliver the chemotherapeutic agent bleomycin to cells with nanosecond-length pulses . These ultrashort pulses can also affect intracellular organelles . Utilizing certain electrical parameters, these nanosecond pulse fields can induce apoptosis to exposed cells . The initial in vitro experiment was carried out using human eosinophils. When the pulses were applied, intracellular granules were modified . In vivo studies have demonstrated that application of nanosecond pulses at an appropriate applied voltage can result in complete regression of the tumor . Similar to irreversible electroporation, nanosecond pulses can be an important tool for clinically treating solid tumors. In addition, these pulses may have potential for applications for gene delivery and for altering cell function.
The parameters for electroporation must be adjusted depending on the characteristics of the molecule that needs to be transferred. A small molecule can pass through the membrane defects by diffusion without any consideration of its charge . However, as molecules increase in size, uptake is not by diffusion alone. For example, DNA does not pass through the membrane by diffusion. Although the exact mechanism of uptake has not been determined, several studies suggest potential mechanisms. One hypothesis is that electrophoretic forces play a role in DNA uptake by cells after EP . Other studies suggest a role of endocytosis in DNA transport . It has also been suggested that DNA can interact directly with the cell membrane when an electric field is applied .
In Vivo Electroporation for Gene Therapy
Gene therapy utilizes nucleic acids instead of drugs to treat disease. As such, the challenge is to efficiently and effectively deliver DNA to the target cells with the least amount of cellular damage and the appropriate levels and kinetics of expression. The methods of gene delivery are divided into two main categories: viral and nonviral delivery. Plasmid DNA (pDNA)-based gene transfer is an attractive approach because it does not require a biological vector and the potential negative effects of viral vectors can be avoided. Plasmid-based gene transfer eliminates the potential of genome integration and environmental spread, and it reduces the potential for unwanted immunogenicity caused by the gene transfer. However, there are limitations to plasmid gene therapy. Low transfection efficiency and low transgene expression and reproducibility are the main concerns. Some of these limitations can be overcome by the plasmid design and the use of chemical or physical delivery methods such as polymer conjugation, particle-mediated delivery, hydrodynamic delivery, ultrasound, and electroporation . The target tissue, the kinetics, and the level of protein expression required for a therapeutic response should be considered when selecting a delivery method.
In vivo EP has emerged as a reliable physical method of delivering pDNA for gene therapy. This method may also be referred to as gene electroinjection, gene electrotransfer, electrically mediated gene transfer, or electrogene transfer. For the purposes of this chapter, “ in vivo EP” is used to describe all these terms. In vivo EP was successfully used to deliver chemotherapeutic agents to tumors in clinical trials for melanoma, squamous cell carcinoma, and basal cell carcinoma . The initial use of this therapy was for the delivery of chemotherapeutic agents to solid tumors . This therapy has been approved for use in several countries of the European Union, and there are published protocols and guidelines for its use in a clinical setting . Preclinical animal studies have been performed using in vivo EP to deliver pDNA to skin, kidney, liver, testis, brain, cartilage, arteries, prostate, cornea, skeletal muscle, heart, and various types of tumors . The increased use of in vivo EP for gene delivery during the past decade enhances its potential for clinical therapeutic applications and is discussed in a number of reviews, book chapters, and journal articles .
In Vivo Electroporation Parameters
A number of factors must be considered when choosing the parameters for in vivo electroporation, including the electrode, the tissue composition, and the actual pulse parameters used. Parameters such as pulse duration, number, magnitude, and frequency are important to the overall effectiveness of the gene transfer . These variables should be optimized based on the tissue type and gene transfer application. The goal is to achieve therapeutically appropriate transgene expression with minimal amount of damage. A number of review articles describe the considerations when using electroporation for gene therapy . Gene expression levels and kinetics can be manipulated for various applications by changing the configuration of the electrodes, the type of electrodes (i.e., plates, needles, or nonpenetrating pins), and electrical parameters.
The electrodes can be divided into two main categories: penetrating and nonpenetrating. Penetrating electrodes are usually an array of varying number of needles—usually two, four, or six—arranged in rows, a grid, or a hexagonal array. The nonpenetrating electrodes do not pierce the skin and are usually two parallel flat plates on tweezers, calipers, or paddles. They can also be composed of multiple electrodes arranged in an array . These electrodes can be purchased from commercial vendors or can be made to certain specifications in the laboratory.
Most pulse generators that are currently used modify the exponential pulse into square pulses. This allows the amplitude of the pulse and the pulse length to be independently controlled. Over time, the design of these pulse generators has been refined, and there are now several commercially available options for experimental and clinical use.
The appropriate field strength is important for efficient gene transfer when using in vivo EP. Typically, the parameters are either high field strength (>700 V/cm) or low field strength (<700 V/cm) coupled with short pulses (microseconds) or long pulses (milliseconds), respectively. Single pulses, multiple pulses, or trains of pulses can be delivered. The frequency of the pulses should also be considered. The target tissue should be considered when selecting the appropriate combination of these variables. In muscle, long low-field-strength pulses produce high expression, but in some cancers short high-field-strength pulses deliver pDNA more effectively . It is generally thought that high-field-strength, short-duration pulses deliver pDNA with low cell mortality, whereas low-amplitude, long-duration pulses yield higher expression but create more damage. Note that the number of pulses can also influence the level of damage for both pulse conditions.
Preclinical Models
Electroporation is increasingly being used as a nonviral gene delivery method because of its high gene transfer efficiency and relatively low adverse effects. It can be used to deliver genes to a variety of tissues such as muscle, the skin, and even directly to tumors. Depending on the delivery target, the choice of electrode, and electroporation parameters used, the level and duration of gene expression can be modulated. A number of studies have investigated EP-mediated gene therapy in nonclinical models. Several review articles have discussed at length the use of EP for targeted gene transfer in preclinical and clinical studies . These previous studies are not described in this chapter. Rather, we discuss more recent studies that utilize EP for gene therapy and have clinical relevance to the treatment of cancer.
In Vivo EP Approaches Using DNA
A number of candidate genes have been the focus of preclinical trials in the effort to formulate gene-based therapies for a variety of cancers. These candidate genes are tumor suppressors, cell growth inhibitors, pro-apoptotic agents, tumor antigens, and immunotherapeutic genes. The therapy can be delivered by intradermal, intramuscular, or intratumoral injection of DNA followed by EP. There is great potential for clinical applications of EP-based gene therapy. Its use in delivering DNA vaccines and RNA for gene silencing is currently being investigated in preclinical models and in clinical trials.
There have been a number of recent developments with regard to the methodology of gene transfer using in vivo EP. Most involve the modification of the tumor microenvironment to enhance transfection efficiency and DNA distribution. One study described the use of an in vitro three-dimensional melanoma model to predict the in vivo transfection efficiency when using EP to transfer DNA . This is a useful tool for reducing the number of animals required for an experiment. With regard to solid tumors, the histological properties play an important role in gene in vivo EP when an external electric field is applied. Soft tumors with large spherical cells, low proteoglycan and collagen content, and low cell density have a higher transfection efficiency than more rigid tumors with small spindle-shaped cells, high collagen and proteoglycan content, and high cell density . This study reinforces the idea that knowledge of the characteristics of the target tissue is important to selection of electroporation parameters. Researchers are trying to develop novel ways to improve transfection efficiency in vivo . Direct modification of the tumor environment by hyaluronidase and collagenase is one way to improve DNA distribution and improve gene transfer . Pretreatment with the enzymes increased the efficiency of gene transfer in tumors with high extracellular matrix content. This effect is less pronounced in tumors with little extracellular matrix. Pretreatment with a hyperosmotic mannitol solution increased reporter gene expression when DNA was introduced by EP in a hind leg tumor model . The authors suggested that this is a result of increasing the extracellular space in the tumor.
Improvement of muscle delivery for cancer therapy has also been a focus of several studies . An oral TLR7 agonist acted as a potential immunological adjuvant in DNA EP vaccination in transgenic tumor models. In a similar study, TLR9 delivered by EP enhanced antitumor effects of a HPV DNA vaccine . EP was used to investigate the role a certain gene plays in tumorigenesis, which can be very useful in designing novel cancer therapeutics. In one study, runt-related transcription factor-1 (Runx1) was overexpressed or knocked down to examine the effects on BCR-ABL-induced leukemogenesis . Alteration of Runx1 expression in BCR-ABL-transformed BaF3 cells affected proliferation and migration in vitro and in vivo .
DNA vaccines delivered by EP are effective in stabilizing cancers. Although this approach has reached clinical trials, additional preclinical studies with alternative genes demonstrate the effectiveness of EP delivery. In a recent study, prophylactic administration of heparanase DNA vaccine in tumor-bearing mice using EP elicited humoral immunity and cytoimmunity and suppressed tumor growth . DNA EP vaccines also retard tumor growth in preclinical studies of breast cancer . A number of studies are using DNA vaccines to treat prostate cancer. EP DNA vaccination with DNA-encoding prostate-specific antigen generated specific responses in vivo . Another study used EP-delivered diphtheria toxin A and prostate-specific antigen in prostate cancer xenografts to slow the growth of the cancer cells . An interesting therapeutic approach is to design a vaccine that contains multiple epitopes that will elicit a diverse immune response. A multi-epitope DNA vaccine against melanoma delivered by EP generated prophylactic and therapeutic antitumor responses .
Angiogenesis is an important process in tumor progression; as such, it is the focus of cancer gene therapy studies. Strategies that can inhibit angiogenesis are of particular interest in anticancer therapeutics. An anti-angiogenic strategy based on in vivo EP of a plasmid encoding soluble vascular endothelial growth factor (VEGF) receptor, a negative regulator of angiogenesis, was the focus of a few studies . A human survivin DNA vaccine delivered by intradermal EP generated a specific cytotoxic T cell response in a mouse model of melanoma . It also suppressed angiogenesis and conferred protection against melanoma. EP of DNA encoding angiopoietin-like 4 (ANGPTL4) to mice did not prevent the growth of primary tumors introduced but caused reduced metastases in the lungs of mice expressing ANGPTL4 .
The transfer of cytokine genes by EP for cancer therapy has been the focus of preclinical studies and even clinical trials. It is intended to generate strong host immune responses that impair tumor growth and lead to long-term immunity. Recent preclinical studies have focused on interleukins and other immune system-related genes . Some studies have used EP to deliver matrix metalloproteinases (MMP) intramuscularly or directly to the tumors to modify them . MMPs are involved in matrix degeneration, tissue remodeling, inflammation, and even metastasis formation; as such, they present an attractive option for cancer gene therapy. In vivo EP of various plasmid-encoded genes (i.e., cytokines and inhibitory molecules) can be combined with radiation therapy or electrochemotherapy as an alternative approach to cancer therapy.
EP Approaches Using RNA
A more recent approach is the use of in vivo EP to deliver RNA to induce RNA interference (RNAi), the downregulation of gene expression produced by sequence-targeted double-stranded RNAs . Two types of RNAs produce RNAi endogenously: small interfering RNA (siRNA) and microRNA (miRNA). These small RNAs are processed in the cytoplasm using a similar pathway. However, the mechanism by which they subsequently downregulate gene expression differs. siRNAs are generally 20–30 nucleotides and complement their target mRNA perfectly. After binding, these RNAs induce cleavage and degradation of a specific target mRNA. miRNAs are often misregulated in diseases or viral infections. miRNAs commonly contain a mismatched base, bind the 3′ UTR of target mRNAs, and repress translation. miRNAs with perfect base pairing to their mRNA target act in a manner similar to siRNAs, causing mRNA cleavage and degradation. Small hairpin RNAs (shRNAs) have a stem-loop double-stranded structure. These RNAs may be synthesized or delivered encoded in plasmids in which RNA expression is generally driven by the RNA polymerase III promoters U6 or H1.
Since 2004, a number of RNAi applications have reached clinical trials for several indications, including cancer therapy . Therapeutically, small RNAs may be delivered as siRNAs, shRNAs, or miRNAs. Synthetic oligonucleotides may be paired to form double-stranded RNA, but they are unstable unless chemically modified on bases, sugars, or in the backbone . RNA delivered from extracellular sources may bind pattern recognition molecules on both immune and nonimmune cells and induce the production of type I interferons, producing immunostimulatory side effects and toxicity . Direct delivery of plasmids encoding shRNAs using methods such as in vivo electroporation may overcome the short half-life of siRNAs.
Gene silencing using in vivo EP as a delivery method was first elucidated in preclinical tumor models using reporter genes . An initial study demonstrated that intratumor EP of plasmids expressing an anti-luciferase shRNA significantly reduced luciferase protein expression in tumors generated with mouse melanoma cells stably transfected with the luciferase gene . This reduction was also observed using in vivo EP of synthetic siRNAs. In a study on B16 mouse melanomas stably transfected with the enhanced green fluorescent protein (EGFP) gene, in vivo EP of siRNA targeting EGFP significantly reduced its expression . EP-mediated intramuscular delivery of siRNA constructs to murine calf muscle reduced the expression of exogenous luciferase for at least 100 days . A similar construct reduced endogenous TLR4 expression for at least 1 week.
Therapeutic in vivo EP of siRNAs or plasmids expressing shRNAs has been tested in several preclinical models for several cancer therapy applications. For these applications, delivery is most often performed directly to the tumor. In a therapeutic demonstration of silencing of a cancer-specific gene, expression of a melanocyte-specific transcription factor, Mitf, was downregulated using siRNA. Delivery to small mouse melanomas induced apoptosis and significantly delayed tumor growth . The addition of interleukin (IL)-12 gene therapy increased this effect . siRNA targeting several apoptotic genes used as adjuvant to plasmid DNA vaccine encoding tumor antigens in spontaneous mouse mammary tumor model significantly produced tumor progression when delivered intramuscularly .
RNAi has been tested therapeutically as a combination therapy with chemotherapeutic agents. In both subcutaneous and orthotopic pancreatic mouse cancer models, intratumor EP of either siRNA oligonucleotides or a plasmid expressing shRNA designed to downregulate the k-ras oncogene significantly reduced tumor growth and increased survival . Combination therapy with the cytosine arabinoside analog gemcitabine significantly increased these effects. In similar combination studies in nude mouse models with subcutaneous human lung carcinoma tumors, the combination of downregulation of the multidrug resistance-associated protein using various siRNAs with the drugs navelbine and epirubicin , which are subject to drug efflux, significantly reduced tumor growth.
Tumor vascularization has been targeted by three groups. In four subcutaneous human tumor xenograft models in nude mice, hVEGF was targeted using modified siRNA oligonucleotides . In the three models with high VEGF expression, a significant effect on tumor growth was observed. In the model with low VEGF expression, no significant effect was observed. Intravenous siRNA injection followed by intratumor EP also effectively slowed tumor growth. Using plasmids expressing siRNAs, the VEGF-A and VEGF-C isoforms were targeted in mouse metastatic mammary cancer . In groups in which the VEGF-A isoform was targeted, a significantly lower tumor volume was observed. Lymph node metastases were reduced after delivery of siRNA targeting either isotype; a reduction in lung metastases required the combination. In a short-term experiment, a GTPase induced by VEGF, Rac1, was targeted in a subcutaneous mouse neuroblastoma model . In vivo EP of targeted, but not control, siRNA significantly slowed tumor growth during a 7-day period.
One group therapeutically tested in vivo EP of miRNA. miR-143 is associated with growth arrest, and levels in prostate cancer inversely correlate with the cancer’s histopathological grade. In vivo EP of miR-143 into subcutaneous human prostate tumors in nude mice significantly slowed tumor growth over a 6-day period .
Although complete tumor regression was not achieved in any of these RNAi protocols, and although only short term (<100 days) tumor growth was monitored, a slowing in tumor growth was observed in each case, indicating that this technique may hold promise therapeutically, either as a sole or combination cancer therapy. However, no clinical trials for RNA delivery as of yet utilize EP.
Translation of In Vivo Electroporation in the Clinic
The ClinicalTrials.gov website ( http://www.clinicaltrials.gov ) is a service of the U.S. National Institutes of Health. It is an extensive database of active clinical trials in more than 170 countries. There are currently 47 clinical trials registered in this database when the search term “electroporation” or “electropermeabilization” is used. Of these studies, 9 have been completed, 20 are recruiting, 5 are active but not recruiting, 2 are enrolling by invitation, 3 have not begun recruiting, 2 have been suspended, 1 has been withdrawn, and the status of 5 is unknown. These trials can be divided into two main categories: those using EP for gene therapy and those using EP for other non-gene therapy approaches ( Figure 7.1 ). There are 18 current non-gene therapy trials using EP. The majority of them are using EP to deliver chemotherapeutic drugs. In these studies, the cancer drug bleomycin is delivered using EP to treat head and neck cancer, colorectal cancer, melanoma, pancreatic cancer, brain metastases, and breast cancer. However, only 4 of these studies are recruiting patients at this time; the remaining studies are suspended, withdrawn, or the current status is unknown.

Two completed trials have examined the safety and tolerability of using EP devices on healthy patients. The MedPulser DNA delivery system (Inovio Pharmaceuticals, San Diego, CA) was used to deliver phosphate-buffered saline intramuscularly to patients . Pain severity was evaluated at the time of EP and at various time points after treatment. The treatment was generally well tolerated, and only two patients reported adverse experiences. The peak pain/discomfort was reported within 5 min of the treatment. The DermaVax electroporation system (Cellectis, Paris, France) was tested for intradermal delivery after the application of a topical anesthetic cream or a placebo cream. The researchers measured pain intensity and skin irritation. Currently, there are no published results from this study.
Three active studies are using irreversible EP to treat liver and pancreatic cancer. They are using the NanoKnife low-energy direct current system (AngioDynamics, Latham, NY) to treat tumors that are not suitable for resection or thermal ablation. These studies are designed with safety and efficacy as the primary outcome measure. It remains to be determined if the success of this technology with animal models is translatable to humans .
Two studies use ex vivo electroporation to introduce pDNA into cells, which are then used to vaccinate the patients. In one study, EP is used to introduce mRNA encoding a tumor-associated antigen into dendritic cells, which are then injected subcutaneously into patients with melanoma. The objective is to boost the patient’s immune response to melanoma. In the other study, autologous cancer cells are harvested from the patients and EP is used to introduce transforming growth factor-β 2 (TGF-β 2 ) antisense/granulocyte–macrophage colony-stimulating factor (GM-CSF) expression vector plasmid. The modified cells are then injected intradermally into the patient. The objective of this study is to increase the expression of tumor antigen and enhance dendritic cell migration to the vaccination site using the GM-CSF transgene while simultaneously reducing the production of immune inhibiting activity by creating a TGF-β2 blockade. Both of these studies are designed with safety, tolerability, and immunogenicity as the primary outcome measure.
Electroporation Gene Therapy for Cancer in Clinical Trials
Clinical trials using EP to deliver pDNA have been the topic of thorough reviews . Twenty-nine studies use EP for gene therapy. Of these, only approximately one-third involve treating cancer ( Table 7.1 ). The remaining studies primarily focus on using EP to deliver DNA as influenza, HIV, and human papilloma virus vaccines and are not discussed here. Of the 10 cancer EP gene therapy studies, 6 focus on therapy for melanoma and the other 4 address colon cancer, Merkel cell carcinoma, prostate cancer, and leukemia. These studies are in either phase I or phase II, and the DNA is injected into the muscle or directly into the tumor.
NCT No. | Phase | Status | Condition | Delivery | Sponsor | Intervention | Outcome measure | n |
---|---|---|---|---|---|---|---|---|
NCT01064375 | I/II | Recruiting | Colorectal cancer | i.d. | Karolinska University Hospital | Biological: tetwtCEA Device: DermaVax (Cyto Pulse Sciences) | Safety and immunogenicity | 20 |
NCT00223899 | I | Completed | Metastatic melanoma | i.t. | Vical | Biological: VCL-IM01 Device: MedPulser (Innovio Pharmaceuticals) | Safety and overall response | 26 |
NCT01440816 | II | Recruiting | Merkel cell carcinoma | i.t. | Fred Hutchinson Cancer Center; National Cancer Institute | Biological: IL-12pDNA | Increased gene expression; safety | 15 |
NCT00859729 | I/II | Unknown | Prostate cancer | i.d. | Uppsala University; Karolinska Institutet; Cyto Pulse Sciences | Biologocal: pVAXrcPSAv53I Device: DermaVax (Cyto Pulse Sciences) | Safety and feasibility of escalating doses; overall immune response | 18 |
NCT00323206 | I | Completed | Malignant melanoma | i.t. | H. Lee Moffitt Cancer Center and Research Institute; National Gene Vector Laboratory | Biologocal: IL-12 pDNA Device: MedPulser (Innovio Pharmaceuticals) | Toxicity and efficacy; recommended dose for phase II study; local and systemic response | 24 |
NCT01502293 | II | Recruiting | Melanoma | i.t. | University of California, San Francisco | Biological: IL-12 pDNA Device: MedPulser (InnovioPharmaceuticlas) | Response rate; overall survival and safety | 25 |
NCT00471133 | I | Completed | Melanoma | i.m. | Ichor Medical Systems; Memorial Sloan–Kettering Cancer Center | Biological: Xenogenic tyrosinase DNA vaccine Device: TriGrid delivery system (Ichor) | Safety and feasibility; antitumor immunologic response | 24 |
NCT01334060 | II | Recruiting | Leukemia | i.m. | Southampton University Hospitals NHS Trust; Imperial College Healthcare NHS Trust; Royal Devon and Exeter NHS Foundation Trust; Innovio Pharmaceuticals; Efficacy and Mechanism Evaluation (EME) Programme; Leukemia Research Fund | Biological: p.DOM-WT1-37 DNA vaccine Biological: p.DOM-WT1-126 DNA vaccine | Molecular response; time to disease progression and survival | 184 |
NCT01138410 | I/II | Recruiting | Melanoma | i.m. | Scancell | Biological: SCIB1 Device: TriGrid delivery system (Ichor) | Safety, tolerability; biological and clinical effects | 22 |
NCT01045915 | I | Recruiting | Melanoma | i.t. | BioAlliancePharma SA | Biological:AMEP | Determination of dose-limiting toxicity | 18 |
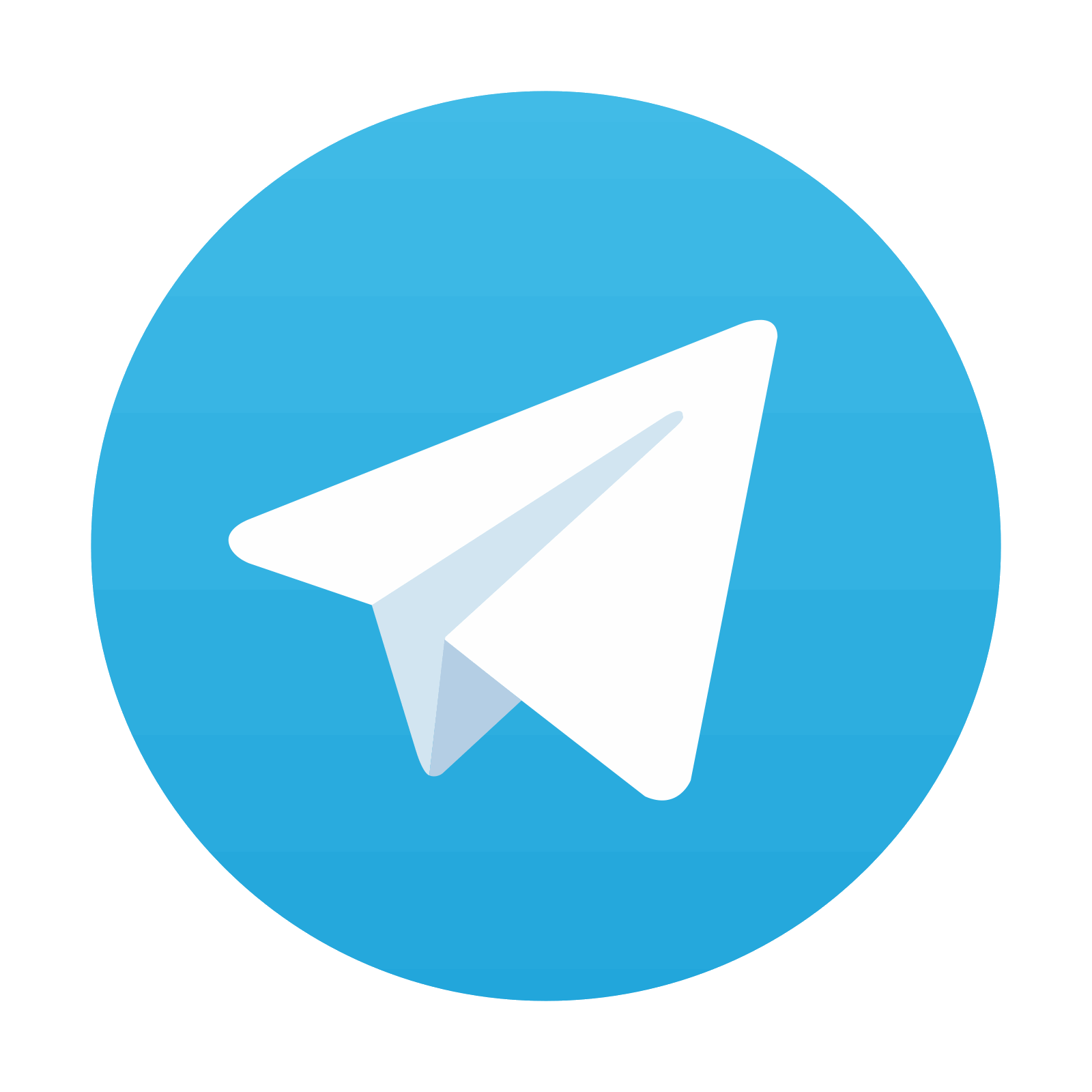
Stay updated, free articles. Join our Telegram channel
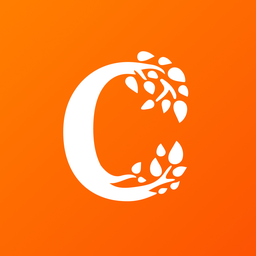
Full access? Get Clinical Tree
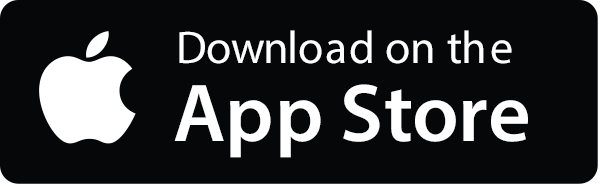
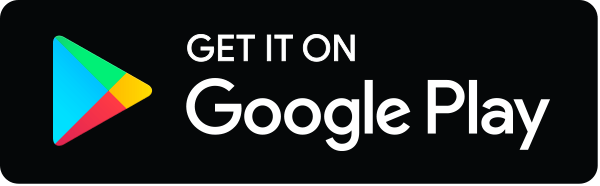
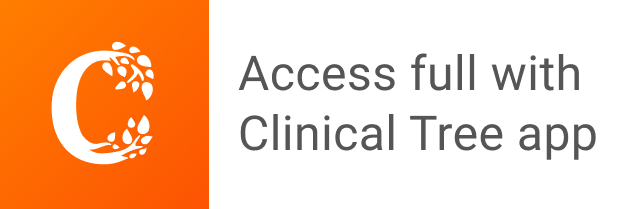