(1.1)
Here μ is the linear attenuation coefficient of the x-rays at point (x, y, z), L is the thickness of the tissue crossed by the x-ray beam, and s is the coordinate along the path of the x-ray beam.
According to Eq. 1.2 (derived from Eq. 1.1),
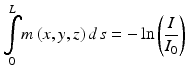
is the line integral of the x-ray attenuation coefficient μ. The line integrals, recorded at different angular position of the measurement system, are the basic measurement parameters in CT. In the image reconstruction process, the local x-ray attenuation coefficients μ are extracted and stored in the image matrix constituted by voxels (pixels in the CT image) with specific coordinates (x, y, z).
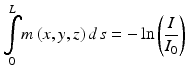
(1.2)
The value μ is characteristic for every voxel of the image matrix, and in SECT it is converted to the Hounsfield Units (HU) value scale according Eq. 1.3:
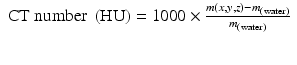
Here, μ (water) is the x-ray attenuation coefficient of water.
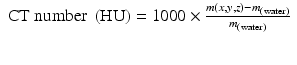
(1.3)
In a single energy CT scan, the technician can adjust different scan parameters in order to obtain an optimal examination, in particular modifying the intensity and the energy of the x-rays.
The intensity of the x-rays depends directly on the tube current of the x-ray tube.
The energy of the x-rays is directly proportional to the differential in potential (ΔV) between the cathode and the anode of the x-ray tube (or in other words it depends on the applied tube voltage). The value of ΔV is expressed in kV.
For high tube voltage, the x-ray beam will be absorbed less in the human body and have higher penetration, and a relatively large amount of x-ray quanta will reach the DS. At a given primary x-ray intensity I 0, image noise will be low. On the other hand, the contrast resolution of the image will be reduced, in particular for CT examinations obtained after intravenous administration of iodine-based contrast agent.
For low tube voltage, the x-rays beam will show higher absorption and less penetration in the human body. The x-rays will interact more with the structures of the human body, and at a given primary x-ray intensity I 0, a smaller amount of x-ray quanta will reach the DS, increasing image noise on the one hand but improving the contrast resolution of the image on the other.
1.3 X-Ray Spectrum and K-Shell Binding Energy
The generation of x-rays in the x-ray tube is a probabilistic process that produces a spectrum of x-rays at different energies. The energy of the x-rays is expressed in keV. The maximum energy of the x-ray spectrum corresponds to the applied tube voltage, its mean energy is directly proportional to it. The spectrum is superimposed by peaks characteristic for the anode material.
A typical x-ray spectrum is shown in Fig. 1.1, and the shape of the curve (and so the x-ray intensity at a certain energy) changes with the applied tube voltage.
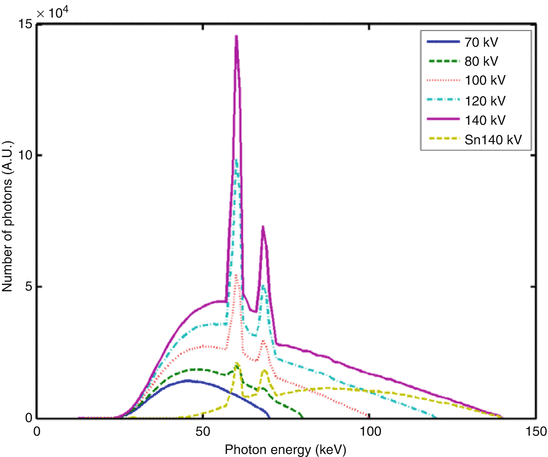
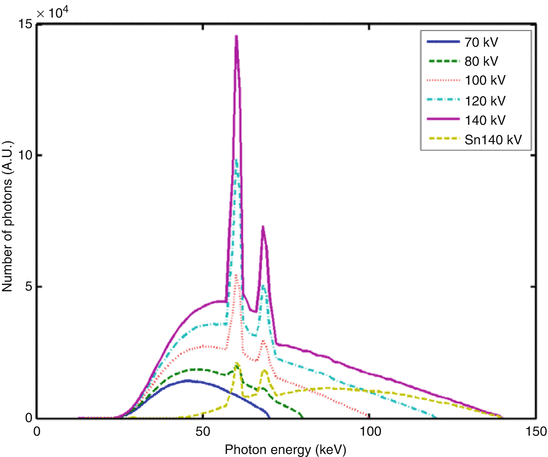
Fig. 1.1
Typical x-ray spectra used in medical CT. The spectra at 70, 80, 100, 120, and 140 kV are obtained after standard pre-filtration. Their mean energies range between 47 and 69 keV. The Sn 140 kV spectrum is obtained after pre-filtration with 0.4 mm Sn (tin) to remove lower energy x-ray quanta and shift the mean energy of the spectrum to higher values
In clinical practice, x-ray tube voltages between 70 and 140 kV are used in CT. For these values, the energy spectrum comprises a range between 30 and 140 keV [1], with a mean energy of about 52 keV for 80 kV tube voltage and 69 keV for 140 kV tube voltage (directly behind the bowtie filter and depending on the pre-filtration of the spectrum) [2].
In this energy range, there are basically two interaction mechanisms of the x-rays with the atoms and molecules of the human body [1, 3, 4]:
Compton scattering: When an x-ray photon hits an electron of the external orbitals (or shells) having a low binding energy, it loses a part of its energy and its trajectory is deflected before reaching the DS, while the electron is knocked out from its orbital. Compton scattering is responsible for a loss of contrast resolution of the images [3]. It predominates in areas of the human body rich in atoms with low atomic number (see below) [5], and it mainly depends on the density of the material [6].
Photoelectric absorption: When an x-ray photon hits an electron of the innermost orbital (noted as K-shell), it loses all its energy, the electron is knocked out from its orbital, and the x-ray photon does not reach the DS [3]. This phenomenon prevails in tissues rich in atoms with high atomic number (see below) [5], and it depends strongly on the x-ray energy and on the atomic number of the material.
The K-shell binding energy is directly proportioned to the atomic number of the respective elements [3]. Elements such as hydrogen (Z = 1), carbon (Z = 6), nitrogen (Z = 7) and oxygen (Z = 8) have a small K-shell binding energy between 0.01 and 0.53 keV, in comparison with calcium (Z = 20, K-shell binding energy 4.0 keV) and iodine (Z = 53, K-shell binding energy 33.2 keV) [2, 3].
The probability of photoelectric absorption is larger if the x-ray energy is similar to the K-shell binding energy [3, 5] and for low values of the x-ray tube voltage [4]. For x-ray energies just above the K-shell binding energy, there is a sudden increase in attenuation, because then the x-ray quantum will lose all its energy to the K-shell electron and will no longer reach the DS. This increase in x-ray attenuation translates into an increase in the HU values of the image [3].
1.4 From Single Energy Computed Tomography to Dual Energy Computed Tomography
In SECT, the scan is performed with a specific x-ray tube voltage according to the characteristics of the patient and the examination type.
For different values of the x-ray tube voltage, the energy spectrum of the x-rays will be different, and x-ray quanta will interact with matter in different ways:
For tissues rich in elements with high atomic number (for example calcium and the iodine of the contrast medium), the mean energy of the x-ray spectrum will be closer to the K-shell binding energy if low tube voltages are chosen. The photoelectric effect predominates, and the HU values will be higher compared with scans at high tube voltage [3–5].
For tissues mainly containing elements with low atomic number, such as hydrogen, carbon, nitrogen, and oxygen (for example fat, or muscles), the K-shell binding energy values are very low and do not differ significantly (from 0.01 keV for hydrogen to 0.53 keV for oxygen). As a consequence, there will be no great difference in attenuation of the x-rays when high or low tube voltages are used [4].
To summarize, the x-ray attenuation μ mainly depends on three parameters:
The elements that constitute the region of interest
The density of the region of interest
The x-ray spectrum for the specific tube voltage ΔV
The x-ray attenuation μ at a specific x-ray energy E can be decomposed into attenuation caused by Compton scattering and attenuation caused by the photoelectric effect, see Eq. 1.4 [7]:

Here μ (x, y, z) (E) is the x-ray attenuation at a specific point (x, y, z).

(1.4)
Using a single x-ray spectrum, two different object regions can have the same attenuation μ even if they differ in chemical composition and material density. As an example, calcified plaques in a vessel can often not be differentiated from the surrounding lumen in the presence of iodinated contrast agent.
Using two different x-ray spectra with two different mean energies E 1 and E 2 in a dual energy CT (DECT) scan can help characterize the material composition of the tissues, because Eq. 1.4 can then be resolved for both μ Compton (E) and μ photoelectric (E).
Moreover, μ (x, y, z) (E) of any material can be decomposed into a linear combination of the attenuation of two base materials 1 and 2, which differ in their photoelectric and Compton characteristics. The relative contributions of these two base materials to each voxel of interest can be determined by measurements with two different spectra [1–5].
1.5 Dual Energy CT Scanners Available in Clinical Practice
In clinical practice, there are several methods to acquire DECT data [2]. Most commonly, two different x-ray spectra are used in combination with standard CT detectors. In commercially available solutions, a variety of different techniques has been introduced:
Performing two subsequent scans with different x-ray tube voltages with a single source CT scanner
Rapidly switching the x-ray tube voltage during the scan
Introducing a split filter into the tube collimator housing of a single source CT scanner
Using dual source CT systems
Another approach uses a single x-ray spectrum in combination with an energy-sensitive detector (dual layer detector).
CT systems with photon counting detectors are still in research state. So far, only pre-clinical prototypes have been available. CT systems with photon counting detectors will therefore not be discussed in detail here.
1.5.1 Scans with Different X-Ray Tube Voltages with a Single Source CT Scanner
This technique has been developed to acquire dual energy data with single source CT scanners without further system modification, such as rapid kV-switching, use of dual layer detectors or split filters in the tube collimator housing [3]. It requires the execution of two consecutive CT scans at different x-ray tube voltages, either in sequential (axial) or spiral (helical) mode. Most commonly, 80 and 140 kV are used, because these are typically the lowest and highest kV settings of a CT x-ray tube which provide best spectral separation, see Fig. 1.2.
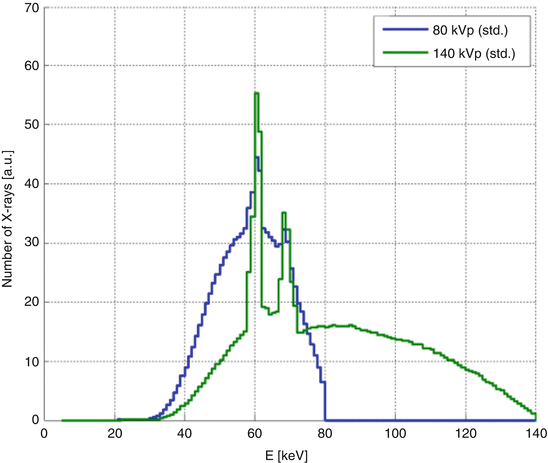
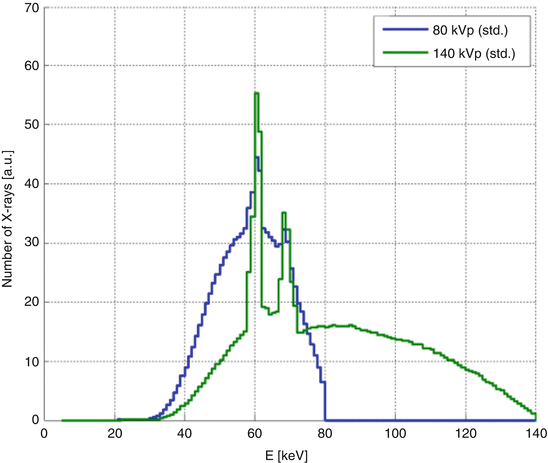
Fig. 1.2
Standard 80 and 140 kV spectra (normalized to equal intensity) used to acquire DE data at two different x-ray tube voltages
Because of the long time delay between the different acquisitions, examinations with administration of contrast agent are difficult, at least in early arterial phases when the contrast density changes rapidly [2, 3].
There are different commercial realizations of this DE acquisition principle [2, 3]:
In the Volume Dual Energy approach (GE Healthcare, Milwaukee, Wis, USA), alternating sequential scans of the same body region are performed at different x-ray tube voltages [3]. Because of the relatively long delay between the sequential acquisitions and the long total scanning time (e.g., 20 s for a single-phase scan of the liver), the Volume Dual Energy technique has been evaluated in prototypes, but never introduced into the market [3].
With increasing z-coverage of the detectors (the z-axis is the patient’s longitudinal direction) and faster gantry rotation time, larger anatomical areas can be covered with one sequential scan, and the delay between subsequent acquisitions at different x-ray tube voltages becomes shorter. Two different vendors offer single source CT scanners with 16 cm detector z-coverage (Aquilion One; Toshiba, Tochigi, Japan and Revolution, GE Healthcare, Milwaukee, Wis, USA), capable of scanning organs such as the heart without table movement. Both systems acquire dual energy data by performing fast sequential scans with two different tube voltages at gantry rotation times of 0.27 s or 0.28 s [5].
Another commercially available technical realization relies on two subsequent spiral (helical) scans of the same body region (Somatom Definition Edge; Siemens Healthcare, Forchheim, Germany), the first one at 80 kV and the second one at 140 kV. As in standard CT examinations, radiation dose to the patient can be optimized by anatomical tube current modulation [3]. Because of the relatively long time delay between the two spiral scans, the use of this technique is indicated for nondynamic examinations that do not require the administration of contrast agent, such as characterization of kidney stones or the examination of tophaceous lesions in patients with gout [3], or for the calculation of mono-energetic images to reduce metal artifacts at a metal-specific high energy. Figure 1.3 shows a clinical example.
Fig. 1.3
Clinical example demonstrating the use of pseudo mono-energetic images to reduce metal artifacts. The DE scan data were obtained using two consecutive spiral scans at 80 and 140 kV
1.5.2 Rapid Switching of the X-Ray Tube Voltage During the Scan
In a more refined approach, the kV-setting of the x-ray tube is rapidly switched between consecutive projections of the same axial or spiral scan. The Discovery 750HD scanner (GE Healthcare, Milwaukee, Wis, USA) is equipped with an x-ray tube and a corresponding generator capable of switching the ΔV peak values from 80 to 140 kV and vice versa in about 0.25 ms during scan data acquisition [1–5] (Fig. 1.4). According to the manufacturer, the CT scanner uses a detection system (Gemstone detector; GE Healthcare, Milwaukee, Wis) characterized by a faster emission of light and shorter afterglow time if compared with standard scintillation detectors [3, 5, 7].
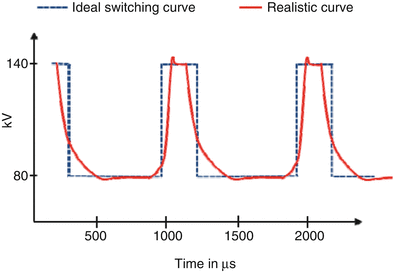
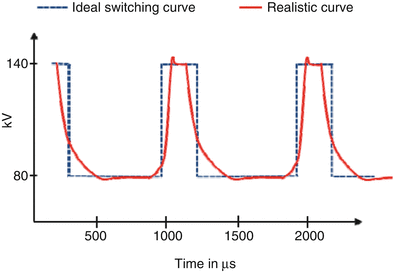
Fig. 1.4
Schematic illustration of rapid kV-switching between 80 and 140 kV tube voltage. Note that two or more low kV projections are acquired for each high kV projection to balance radiation dose
One advantage of rapid kV-switching is the almost simultaneous acquisition of low-energy and high-energy projections with a time delay of less than 0.5 ms, which prevents registration problems due to organ motion or contrast agent dynamics. Dual energy scan data are acquired in the full SFOV of 50 cm diameter [3, 5].
As a downside, fast switching of the x-ray tube current simultaneously to the fast switching of the x-ray tube voltage is technically not possible, and at equal x-ray tube current the x-ray flux at 80 kV is much lower than at 140 kV [2, 3, 5]. To balance the radiation dose of the low kV and the high kV data, two or more low kV projections are acquired for every single high kV projection, see Fig. 1.4. As a consequence, however, the total number of high kV projections during one rotation is reduced, resulting in potential sampling problems and limiting the maximum achievable spatial resolution. As of today, optimizing the radiation dose to the patient by using anatomical dose modulation is not possible. The potentially increased overall radiation dose can at least partially be reduced by combining DE data acquisition with the use of iterative reconstruction (Adaptive Statistical Iterative Reconstruction [ASIR]; GE Healthcare, Milwaukee, Wis) [2, 3, 5]. As another disadvantage, spectral separation cannot be improved by introducing separate pre-filtration of the low kV and the high kV beam (see below). As a practical clinical limitation, the manufacturer provides only images from the 140 kV data set and not from the 80 kV data set [3]. Figure 1.5 shows a clinical example.
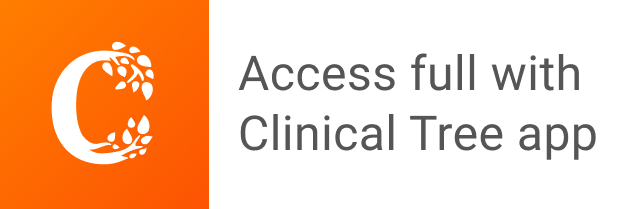