Keywords
Dendritic cell vaccination, Major histocompatibility complex (MHC), CD11c + myeloid and CD11c − plasmacytoid dendritic cells, Clinical trials, Transfection, Viral transduction, Tumor cell hybrids, Pathogen-associated molecular patterns (PAMP), MHC Class I Pathway, PAMP Stimulation, Rational combination therapy
Introduction
Vaccination has been very effective in inducing protective immune memory, preventing or limiting clinical illness from infection. Acellular vaccines essentially target dendritic cells (DCs) in vivo with antigen (the specific target of the required immune response) combined with adjuvant (inorganic or pathogen derived material) that induces DC immunogenic function. Some infectious agents and most cancers have proven to be difficult targets for acellular vaccination, and vaccination against an existing disease burden has proven a greater challenge.
DCs comprise diverse subsets sharing common properties. Typically, DCs efficiently acquire exogenous antigen, presenting this on major histocompatibility complex (MHC) class II and cross-presenting onto MHC class I. They express high levels of MHC and costimulatory molecules. They can reside in many tissues but migrate to secondary lymphoid tissue where their characteristic morphology of folds and dendrites promotes multiple simultaneous contacts with co-localizing T cells. They interact with a wide variety of immune cells, critically link components of innate and adaptive immune responses, and can activate de novo and recall T lymphocyte responses.
This chapter focuses on DCs as vaccines, but it is important to recognize that B lymphocytes and macrophages may also be considered as professional antigen-presenting cells (pAPCs). B lymphocytes selectively endocytose the cognate antigen for their surface immunoglobulin and can present this on MHC class II. Macrophages express MHC and efficiently clear particulate and soluble complex molecules, but unlike DCs, they prioritize proteolysis over epitope preservation. Other cell types, such as γδ T cells, may possess pAPC properties, but these have not yet been used as vaccines in trials .
The types of human DCs and their relationship to cellular vaccines have been extensively reviewed . This chapter focuses almost entirely on monocyte-derived DCs (moDCs) or CD34 + progenitor-derived DCs that have been cultured ex vivo . The culture process is exploited both to achieve DC differentiation and to enable manipulation of immune-stimulatory function so they present epitopes to T lymphocytes and provide costimulation through cell surface and secreted molecules. These approaches have the advantage that many millions of DC vaccines (DCVs) can be generated through leukapharesis or by selection of monocytes by plastic adherence or CD14 selection from relatively small peripheral blood samples.
In addition, CD11c + myeloid and CD11c − plasmacytoid dendritic cells (mDCs and pDCs, respectively) can be directly isolated from peripheral blood. pDCs (~0.1% of blood mononuclear cells) express a repertoire of characteristic markers that do not include typical myeloid nor lymphocytic lineage markers. Inflammatory stimuli result in their developing the typical dendritic morphology, antigen-presenting function, and, in particular, release of large amounts of type 1 interferons. mDCs express myeloid markers CD13 and CD33 and also MHC class II. These can be further divided into subpopulations according to expression of CD1c, CD16, and BDCA3. Of these, the most abundant are the CD16 + mDCs, whereas the CD1c + population may be the most effective stimulators of T cell responses.
The generation of moDCs typically requires differentiation of monocytes in granulocyte–macrophage colony-stimulating factor (GM-CSF) and interleukin (IL)-4 (reviewed in Banchereau ) or, alternatively, IL-15 . A critical DC property is encapsulated in the paradigm of “maturation,” in which DCs can be stimulated to undergo radical phenotypic change through cytokine signaling and recognition of microbes and injured cells. Further change, termed “licensing,” is brought about by interactions with other activated immune effectors. Together, these initiate varied patterns of altered expression in approximately 6000 genes . Importantly, so-called immature DCs are also functionally active and appear, for example, to contribute to peripheral antigen tolerance by deleting autoreactive T cell clones and inducing antigen-specific regulatory CD4 T cells .
An increased understanding of DC function, particularly in terms of antigen uptake, now serves as a model for the development of rationally designed acellular vaccination specifically targeted at physiological DCs in vivo . However, this chapter focuses on ex vivo -generated cancer DCVs, which can be regarded as engineered therapeutic constructs, derived from human cells and modeled on their physiological counterparts. Their usefulness derives from the potential to characterize and modify multiple immune parameters in vitro , and these may yet prove a useful therapeutic strategy.
DC Vaccines Used in Clinical Trials
A very large number of clinical trials of DCVs for cancer have been carried out across a wide range of malignancies. Meta-analyses have been undertaken on types of DCVs and outcomes in specific tumor types—melanoma and renal and prostate cancer—that serve to illustrate the major issues arising in this field. Twenty-nine evaluable trials were identified that used autologous DCVs to treat renal or prostate cancer . These included 906 patients. Most were single-arm phase I or phase II trials including 6–35 patients, but interestingly, 3 randomized trials testing the clinical efficacy of a DCV preparation with or without antigen loading in prostate cancer were included . Nakai et al . reviewed 54 clinical trials of DCVs including 967 patients with melanoma published over a decade . This included a single randomized trial testing the response rate induced by DCV vaccination versus conventional chemotherapy for people with advanced melanoma .
Demonstration of clinical benefit for a cancer vaccine requires evidence of prolonged survival or, as a surrogate, progression-free survival, or improved quality of life and survivorship for an intervention that has equivalent time-to-event outcomes as standard treatment. In this regard, the first phase III randomized trial to be reported was disappointing. Patients with advanced melanoma ( N =108) were randomly allocated treatment with DCV versus standard chemotherapy. The trial closed at interim review because there was a very low probability of detecting a signal of efficacy in terms of response rate, progression-free survival, or overall survival. The DCV product design was prototypical for most contemporary trials. Autologous moDCs were derived from peripheral blood mononuclear cells (PBMCs) by plastic adherence followed by differentiation in GM-CSF and IL-4 and maturation in tumor necrosis factor-α (TNF-α), IL-6, IL-1β, and prostaglandin E 2 (PGE 2 ). These were loaded with a cocktail of peptides derived from melanoma antigens appropriate to the patients’ MHC alleles . Sipuleucel-T is a different product. An autologous leukapharesis product is enriched for DC precursors by a two-step differential centrifugation process, and the resulting preparation still contains predominantly T cells as well as monocytes, natural killer cells, and B cells. Although not specific for DCs, the adhesion molecule ICAM-1 (CD54) was demonstrated to be a marker for pAPC: CD54 + cells comprise on average less than 15% of the cells. These are exposed to PA2024, a fusion protein of tumor antigen, prostatic acid phosphatase (PAP), and GM-CSF . Four independent trials have reported consistent results . The largest randomized 512 men with advanced refractory prostate cancer to sipuleucel-T or the same cell preparation with no PAP–GM-CSF for three intravenous infusions of greater than 4×10 7 cells every 2 weeks. This resulted in a 4.1-month prolongation in median survival and a 22% reduction in hazard of death over a median 34 months of follow-up.
Few other randomized trials of DCVs have been reported, and all are small (<30 participants) . They include one indicating that immature DCVs were no better than an acellular vaccine and another that rationalizing selection of intranodal over other vaccination routes . The vast majority of DCV trials for both urological cancers and melanoma have exploited moDCs differentiated from PBMCs through exposure to cytokines . A smaller number have used CD34 + precursors as a source of DCVs. The source of cytokine-cultured DCVs does not associate with the clinical response rate .
Importantly, immune therapies can cause transient increases in tumor size from immune infiltration , and this has been clearly demonstrated in at least one DCV trial . It can be argued that mixed responses (MRs) and stable disease (SD) may represent signals of efficacy in phase II trials of immune therapy and that combining all these groups in the clinical benefit rate (CBR) is meaningful. Even so, this remains problematic, particularly because of varying and imprecise definitions of SD . In meta-analysis, DCVs against prostate cancer in 181 patients resulted in an overall response rate of 7.7% and CBR of 54%. Of 166 renal cancer patients, DCV treatment induced objective responses in 12.7% and the CBR was 48% . In melanoma, the mean response rate in more than 900 patients in 53 trials was 7%, with a CBR of 23% .
In a meta-analysis of DCV trials for urological cancers, cell-mediated immune responses to DCVs have been reported using at least one assay in 77% of 256 prostate cancer patients and 61% in those with renal cancer . Importantly, detection of cellular immune response to DCVs increased the probability of clinical benefit—that is, response or stable disease in both renal and prostate cancer patients. Similarly, in a systematic review of multiple DCV trials for people with advanced melanoma, a measurable increase in the number of circulating interferon-γ (IFN-γ)-specific T cells was reported in 161 of 280 patients (58%). Delayed-type hypersensitivity to cutaneous antigen challenge test was observed in 164 of 359 patients (46%). In both cases, a possible association of immune with clinical response was observed . These estimates are threatened by multiple sources of bias but nonetheless suggest that cell-mediated immunity is routinely observable in DCV trials and meaningful in vaccine development, such as in comparing vaccines or combination strategies.
In the randomized trials of sipuleucel-T versus a placebo cell preparation, titers of tumor antigen-specific antibody served as a marker for helper T cell induction: A greater than fivefold increase was observed in 28 versus 1% of the respective cohorts. More directly, an increased tumor antigen-specific T cell proliferation was detected in 28 versus 8% of patients. A vaccine-induced antibody response, but not a T cell proliferation response was associated with better survival .
A wide variety of assays have been used in DCV trials, and details on their conduct and full disclosure of results are commonly lacking in published reports. Further development of this field depends on adopting by consensus a more uniform and complete reporting framework for T cell assays, incorporating information regarding the laboratory environment, sample acquisition and processing, the assay, and the generation and analysis of the data. This comprises a minimum information data set for a tumor vaccine trial .
The further development of novel DCV technology through trials faces a number of challenges in terms of trial design . Unlike conventional anticancer pharmaceutical agents, DCV doses cannot be selected using pharmacokinetic criteria because vaccination depends on a secondary effect mediated through immune amplification. Phase I trials of cytotoxic agents are modeled on a direct dose–effect relationship in which acute toxicity is a surrogate measure of tumor killing, but this does not apply to DCV trials. DCV strategies may be most effective in delaying or preventing progression in the setting of minimal residual disease following other modalities rather than in directly shrinking bulky tumors with an immunologically hostile microenvironment. Thus, relying solely on tumor response rates may wrongly reject potentially valuable DCV strategies. For these reasons and with a large amount of safety data already available for cancer DCVs, future phase I trials need to be constructed to demonstrate proof of biological principle for the doses or strategies being used. Evidence of immunogenicity serves as an intermediate outcome measurement. Phase I trials should include cohorts sufficiently large so as not to miss immune effects by chance, and there is a strong case for randomizing between treatment doses or strategies to demonstrate real technological advance.
Engineering Antigen Uptake in DCVS
Overview
Loading of vaccine-grade DCs with antigen is a critical step in the production of an immunogenic vaccine. Exogenous approaches include individual peptide epitopes, partially preprocessed “long” peptides , and use of recombinant proteins or tumor cell lysates and apoptotic tumor cells. However, antigen can also be expressed endogenously within the DCs by transduction with recombinant viruses, transfection with synthetic nucleic acids, and use of dendritic cell–tumor cell hybrids. The topography of the p-MHC following direct binding of free peptides at the cell surface may be qualitatively different from the presentation of the same sequence derived by antigen processing and loaded in endosomes or the endoplasmic reticulum (ER) . MHC II complexes may be generated more efficiently from endocytosed material than from peptide pulsing . Endogenous production of target antigen means that prior knowledge of epitope sequences and MHC restrictions is not required and recruitment need not be limited by HLA type. Endogenous antigen expression bypasses antigen uptake mechanisms. The antigen-encoding sequence can be modified to include signals selectively targeting it for processing and can be delivered alongside additional sequences to optimize immunogenicity . Endogenous antigen expression may result in more prolonged epitope presentation and more effective T cell stimulation than external peptide loading . An important observation is that maximal obtainable peptide–MHC class I surface densities are not necessarily associated with optimal T cell stimulation: Low to intermediate surface peptide–MHC density levels in DCVs in vivo may be more effective in inducing cell responses and higher avidity immune memory. The mechanism could include shedding of surface peptides at high densities, leading to tolerogenic presentation to T cells, or activation-induced death of high-avidity T cell clones . Even low levels of antigen expression in DCVs may be immunogenic.
Transfection
Despite early reports , transfection using plasmid DNA and cationic lipid-based transfection have been almost uniformly unsuccessful with human DCs , as with most primary, nondividing cells with antigen expression in at best 10–20% cells and impairment of viability . Particle bombardment of plasmid DNA into human DCs led to efficient stimulation of melanoma-specific T cell responses of multiple specificities in vitro , enhanced by cotransfection with IFN-α or IL-12 DNA . Plasmid emulsified with a cationic peptide (CL22) was actively taken up by highly phagocytic immature DC in a protocol including transient chloroquine exposure to enhance lysosomal egress of intact DNA. This system exhibited up to 55% transfection efficiency in human monocyte-derived DCs , and presentation of MHC class I-restricted viral epitopes induced T cell recognition in vitro . In a mouse model, vaccination induced CD8 + responses and opsonizing antibodies, suggesting induction of helper T cells. In a phase I/II trial, this method was used to transfect immature moDCs from patients with metastatic melanoma with a plasmid encoding two melanoma differentiation antigens in tandem (gp100/PMEL17 and Melan-A) before maturation and vaccination. Circulating T cell responses were demonstrated to MHC class I and class II epitopes as well as whole antigen, and clinical benefit was demonstrated in several patients. Interestingly, levels of whole antigen expression in DCVs were highly variable and did not associate with clinical outcome. Even DC preparations expressing very low levels of whole antigen could be positive for HLA-A2 restricted surface epitopes .
In principle, RNA might be more efficient than the use of plasmid DNA by removing the requirement for nuclear access and the transcription step. Furthermore, whole tumor RNA can be used for transfection, broadening the range of targets compared to sequences encoding defined tumor antigens . However the cellular half-life of RNA is thought to be short and transient antigen synthesis may not be ideal for the generation of highly avid T cell effectors. Also, the translation efficiency of in vitro transcribed RNA and the stability of its interaction with ribosomes depend on the length of poly-A tails, which can be difficult to control in vitro , particularly if RNA is synthesized and then polyadenylated. There is evidence that incorporation of poly-A sequences into the template DNA is more reliable and robust and leads to higher levels of transgene expression, and that at least 120 adenosine nucleotides are required . Immunogenicity of RNA-transfected DC vaccines can be maximized by manipulation of noncoding flanking sequences and the nature of the 5′-methylguanosine cap .
Although initial reports of DCs transfected by passive pulsing and lipid-mediated transfer of in vitro transcribed RNA were promising, electroporation of RNA appears to be the optimal method for transfection of DCs , leading to high sustained transgene expression levels without compromising viability . RNA-transfected DCs are immunogenic in vitro for both CD8 + and CD4 + T cell responses, and this transfection platform has been tested clinically for patients with prostate , central nervous system , and renal cell cancer and malignant melanoma .
If the DCs are required to actively take up nucleic acid, “immature” highly phagocytic DCs seem optimal, whereas electroporation allows introduction of nucleic acid regardless of phagocytic capability. Transfection before maturation stimuli may lead to an early and less immunogenic peak in epitope display, whereas transfection after maturation may allow peak epitope presentation to coincide with DC arrival in secondary lymphoid tissues, resulting in stronger and longer T cell stimulation . However, it was also reported that although transfection after maturation led to increased early transgene expression, increased yield of transfected cells, a more stable “mature” phenotype, increased cell motility, and generation of stronger IFN-γ responses from CD4-restricted T cell clones, the ability of transfected DCs to expand Melan-A 26–35-specific CD8 T cells within an autologous system did not vary according to whether transfection was performed before or after maturation .
Viral Transduction
Viral transduction of DCs can be highly efficient in terms of transgene expression and the proportion of target DCs expressing the antigens of interest . Comparisons suggest that viral transduction is far more efficient than nucleic acid (particularly DNA)-based approaches in this regard , although the superiority of viral transduction can be dependent on the nature of the target DCs: RNA electroporation was equivalent to adenoviral transduction in CD34 stem cell-derived DCs . There is evidence that viral transduction may preserve the ability of the tumor-associated antigen-expressing DCs to secrete IL-12p70 compared with RNA transfection .
However, it can be very time-consuming to manufacture recombinant viruses, and these have several disadvantages for clinical use, including the possibility of insertional mutagenesis and the possibility of neutralization of the DC vaccine by cellular and humoral immune responses directed against viral antigens rather than the introduced tumor-associated-antigens . There may also be competition between immunodominant viral epitopes and less immunogenic, self-tumor antigen epitopes.
Viral transduction can compromise DC antigen presentation via interference with MHC class I processing machinery, including TAP (transporter associated with processing) function, reduction in inflammatory cytokine production, and direct cytotoxic effects on DCs , due to well developed viral immune escape mechanisms are well evolved. For example, the adenovirus E3 protein limits MHC class I presentation by sequestering class I molecules in the ER , and adenovirus E1A protein inhibits immunoproteasome function .
Most viral vectors can be engineered to contain relatively long inserts of foreign genetic material (up to 25 kb) , and this allows simultaneous expression of multiple tumor-associated-antigens, offering some protection against the development of antigen loss variants within tumors. In contrast, RNA electroporation of DCs becomes less efficient the greater the size of the mRNA used.
Retroviruses require cell division to express viral transgenes; therefore, this approach is limited to transduction of CD34 hematopoetic stem cells followed by differentiation to mature DCs because mature, terminally differentiated DCs are resistant to transduction. With this approach, however, only 25% of cells differentiated to DCs, and of these, 25% expressed the transgene (Melan-A), although these DCs were able to drive Melan-A-specific responses within autologous peripheral blood lymphocytes, after two stimulations .
In a systematic comparison of various viral vectors, it was found that generally transduction of immature DCs was most efficient and that adenoviral efficiency was the highest, with lentivirus being the least efficient. Adenoviral transduction also led to increases in markers of phenotypic DC maturation, such as CD83 and CD40, to a greater extent than other viruses, although all viruses upregulated type 1 interferon and IFN-γ release from DCs. Retroviruses and lentiviruses appear to have relatively low intrinsic immunogenicity compared to adenovirus, whereas adenovirus has the least cytopathic effects of the DCs.
A number of trials have used virus-transduced DCVs, generally reporting a weak signal of clinical efficacy and evidence of immunogenicity for CD4 + and CD8 + effectors. In the setting of metastatic melanoma, such reports include vaccination with moDCs transduced with replication-deficient adenovirus expressing Melan-A , the use of CD34-derived DCs transduced in the immature state with a modified vaccinia Ankara virus encoding human tyrosinase , and the use of DCs transduced with adenovirus expressing gp100 and Melan-A .
In a murine model, an autologous bone marrow DC vaccine transduced with a lentiviral vector encoding three hepatocellular carcinoma-associated antigens gave protection against tumor challenge and also retarded the growth rate of preexisting hepatocellular tumors. Vaccination was associated with a brisk tumor-infiltrating lymphocyte response and with measurable tumor-specific T cells .
In the preclinical setting, human immature moDCs transduced with a recombinant lentivirus encoding the MAGE-A3, in the immature state, were able to present a class I and class II restricted epitope to specific T cell clones, although levels of recognition were four- to fivefold lower than that of target cells loaded with cognate peptide . Epitope presentation by these DCs was also demonstrated by their ability to prime MAGE-A3-specific CD4 and CD8 responses within an autologous peripheral blood lymphocyte population.
DCV–Tumor Cell Hybrids
Advantages of vaccines based on DC–tumor cell hybrids include likely persistent antigen presentation, access to a wide repertoire of tumor antigens, access to the DC antigen processing machinery, and the requirement for only small amounts of tumor tissue to prepare tumor cells. In a murine model, vaccination with polyethylene glycol-induced B16–DC fusion hybrids led to a far superior rate of protection against subsequent challenge with B16 melanoma xenografts compared to B16 melanoma cells alone or a mixture of B16 cells and bone marrow-derived DCs . The use of polyethylene glycol to achieve cell fusion can be toxic to cells and results in relatively low fusion efficiency. Electrical methods of fusion have been developed and lead to presentation of class I and class II restricted epitopes in the murine setting . Hybrid DC vaccines have been used in the clinical setting for treatment of refractory metastatic melanoma. The fusion partners to monocyte-derived DCs were primary autologous tumor cells, and subcutaneous admiration of the vaccine was very well tolerated. A small proportion of patients (3 of 17) derived some clinical benefit from vaccination in the form of a mixed response, disease stabilization, and one partial response at all metastatic sites . In 18 evaluable patients with renal and breast cancers, vaccination with a hybrid DC preparation resulted in evidence of immunogenicity in 10 patients for CD4 + responses and 7 patients for CD8 + cells. Disease regression was seen in 2 patients. Critically, however, in nearly half the patients, inadequate numbers of tumor cells were obtained and vaccine generation was unsuccessful .
Antigen Processing in Dendritic Cells
Different cell types vary in protein degradation processes and the presentation of epitopes. For this reason, understanding these pathways in DCs generally and within specific types of DCVs is important in engineering effective vaccines. These pathways represent potential targets for manipulation.
MHC Class I Pathway in DCs
The MHC class I pathway is active in most cell types, exposing endogenous antigen to immune surveillance as a defence against intracellular, particularly viral, infection . Proteasomal hydrolysis leads to generation of polypeptides 8–20 amino acids in length. Most proteasomal substrates are not full-length, senescent proteins but, rather, defective ribosomal products (DRiPs) . Protein synthesis is linked to presentation of MHC class I-restricted epitopes , and newly synthesized proteins are degraded, in a proteasome-dependent manner, within minutes of synthesis . Conversely, destabilization of antigen proteins by amino acid substitutions increasing vulnerability to proteases did not always increase the supply of MHC class I ligands . Although misincorporation of amino acids and premature protein truncation lead to DRiP generation, specialized immunoribosomes may exist for a similar purpose . Proteasomal products are further trimmed by cytosolic aminopeptidase enzymes, following which there is a limited time period for interaction with MHC molecules. As a result, the vast majority of proteins and peptides are immunologically irrelevant because only a minute fraction bind tightly with class I molecules. Cytosolic peptides are actively transported to the ER to bind nascent MHC class I by a multisubunit ER membrane complex including TAP, tapasin, chaperone protein Erp57, and calnexin .
Within DCs, activating, maturation-inducing signals—particularly signals via Toll-like receptors such as lipopolysaccharide (LPS)—induce rapid and transient formation of DC associated aggresome-like induced structures (DALIS). These are aggregates of polyubiquitinated newly synthesized defective ribosomal products, with localized clustering of ribosomes . These structures seem unique to DCs and macrophages . DALIS may delay presentation of class I-restricted peptide epitopes for 24–36 hr to allow coordination of the arrival of the DCs in the T cell zones of lymph nodes and epitope presentation to T cells . In addition, the sequestration of DRiPs in DALIS may act as a “cellular sink” for antigen and be permissive for cross-presentation of endolysosomal antigens on MHC class I molecules .
The nature of the maturation stimulus can have profound effects on protein translation and subsequent CD8 T lymphocyte recognition. For example, in DCs transfected with RNA encoding tumor antigens tyrosinase, gp100, and CEA, subsequent exposure to Toll-like receptor (TLR) agonist polyinosinic–polycytidylic acid (poly I:C) in the maturation cocktail resulted in reduced antigen expression and epitope presentation , possibly through activation of innate antiviral effector molecules, limiting the rate of translation of viral antigens.
Dendritic cells express immunoproteasomes as well as standard proteasomes , defined by the substitution of LMP2, LMP7, and Mecl-1 for the β 1 , β 5 , and β 2 subunits . Immunoproteasomes alter substrate specificity, preferentially cleaving after basic and hydrophobic amino acids rather than acidic ones . For many viral antigens, epitope generation and binding stability to class I molecules are enhanced by the presence of the immunoproteasome. However, this is not always the case for tumor antigens; in fact, several immunodominant epitopes from tumor-associated antigens are generated rather inefficiently by immunoproteasomes . Indeed, in RNA-transfected human moDCs, downregulation of immunoproteasome function using small interfering RNA enhanced generation of HLA A2-restricted cytotoxic T cell responses to epitopes within MAGE-A2, gp100, and tyrosinase .
Protein ubiquitination is necessary for tagging of proteins for proteasomal processing, and this has been exploited to design “optimized” DCVs against tumor-associated antigens. Using murine bone marrow-derived DCs transfected with RNA, cotranslational expression of ubiquitin upstream of the antigen led to enhanced priming and expansion of tumor-specific CD8 T lymphocytes due to increased rates of antigen degradation . Class I presentation by transfected DCVs can also be enhanced and optimized through the cellular localization and topology of the expressed antigen. The immunodominant HLA-A2-restricted epitope within Melan-A is located in the transmembrane domain of the protein. Rearrangement of membrane topology leads to relocalization of the protein from predominantly the Golgi apparatus to the ER, and this leads to increased sensitivity to lysis by a CTL clone specific for the epitope . Expression of preprocessed polypeptides containing immunologically relevant epitopes alongside an ER targeting/retention signal sequence and coexpression of antigens with “optimal” proteasomal substrates or ubiquitin have been attempted to boost class I presentation .
The use of debris from lysed tumor cells or whole killed cells as the antigen source enables the targeting of multiple shared antigens without preselection or characterization of the antigen. Although conventionally exogenous antigen is considered a source of MHC class II binding epitopes, DCs can also cross-present endocytosed antigen on MHC class I. This is an important property for cancer DCVs that must activate CD8 + T cell responses capable of recognizing endogenous tumor-specific antigens on malignant cells. Furthermore, the simultaneous presentation of antigen on MHC class I and class II supports the generation of coordinated immune responses and the licensing of CD8 + cells by activated CD4 + cells. The pathways of access to MHC class I loading include egress of antigen from endosomes, proteasomal processing and TAP-dependent cytosolic transit to the ER, as well as TAP-independent cross-presentation, which can occur by direct loading of antigen onto MHC I within endosomes .
The MHC Class II Processing Pathway in DCVs
Endocytosed proteins, via any mechanism, as well as endogenous cytosolic proteins and the products of autophagy, access MHC II predominantly via endosomal mechanisms, although access to the Golgi apparatus or ER is also described. The principles of organization of the endosomal compartment include the recycling of endocytosed cell surface receptors through rapid and slow pathways, sorting of endocytosed material, and the progressive exposure of acquired antigen to low pH and proteases within lysosomes. In DCs, MHC class II is localized within lysosomes, bound to trimeric Ii complexes. Progressive cleavage of Ii results in generation of the CLIP nonamer, which is displaced from the MHC peptide binding groove by sequences within antigen. Lysosomal proteases also cleave antigen, unlocking the epitopes bound to MHC. Importantly, lysosomal proteolysis is less efficient in DCs than in other cell types, including through the expression of inhibitors at this location. The reduced protein degradation enables the retention of epitope sequences and indeed permits DCs to continue to generate new MHC–peptide complexes for several days after initial exposure. Immature DCs accumulate abundant MHC class II in the lysosomal compartment but express low levels on the cell surface. This associates with strong endocytic activity. Maturation, in response to stimuli including pathogen-associated molecular patterns (PAMP), cytokines, and interaction with T cell ligands, describes a near complete cessation of macropinocytosis and transport of MHC class II complexes from the lysosomes to the plasma membrane. There is an enhanced ability for MHC class II to form complexes with previously internalized antigen . These coincide with cytoskeletal changes resulting in the formation of folds and dendrites, greatly increasing the surface area for T cell contact.
For all approaches leading to endogenous production of antigen within the target DCs, it is expected that there would be preferential channeling of resultant peptide epitopes into the MHC class I pathway, leading to predominant CD8 and CTL T cell responses. For the purposes of vaccination against malignant disease, this would be a suboptimal outcome because CD4 helper T cells play key roles in antitumor immunity . In attempts to enhance MHC class II epitope presentation after gene insertion into DCVs, endogenous tumor antigen sequences have been modified to drive ectopic localization in the endolysosomal system. Such “tags” include lysosomal-associated proteins such as LAMP-1 or the cytoplasmic domain of the human invariant chain. These lead to efficient generation of class II-restricted epitopes without compromising class I presentation. Both LAMP-1 and invariant chain have been used in the clinical setting . In a model system in mice, preexposure of DCs to antisense oligonucleotides to “knock down” invariant chain expression followed by RNA transfection with antigen-encoding sequences resulted in increased MHC class II epitope presentation, presumably through MHC class II loading in the ER. However, numerous preclinical studies have demonstrated that unmodified tumor antigens, endogenously expressed after RNA transfection of viral transduction, gain access to MHC class II processing and presentation . Specific endolysosomal targeting sequences were identified within the native sequence of the melanocyte lineage antigen, gp100, critical for generating CD4 + T cell responses . This may vary between antigens: Melanosomes and endolysosomes share several structural and enzymatic features that may explain the natural affinity of melanosome antigens for the class II pathway . Macroautophagy is a bulk, nonselective process of self-digestion of senescent cellular proteins, providing a reserve of constituent amino acids during nutrition deprivation and cellular stress. Autophagosomes fuse with endolysosomes and deliver their cargo to the class II loading compartments . In DCs, macroautophagy is a constitutively active process, and the class II loading compartments of in vitro cultured DCs continuously receive input from autophagosomes . Inhibition of macroautophagy has reduced levels of class II epitope presentation from numerous endogenously expressed antigens, including model antigens , viral antigens , and tumor antigens . It is estimated that 20–25% of all class II-restricted peptides are in fact derived from cytosolic or nuclear, as opposed to membrane or extracellular, proteins .
Antigen Transfer between DCVs to Tissue Resident DCs
It is commonly assumed that DCVs migrate from the injection site to T cell zones of lymphoid tissues, where they directly present MHC-restricted epitopes. However, evidence from a murine cutaneous HSV infection model suggested that lymph node resident DCs acquire antigen “exogenously” from migratory DCs and then cross-present it to CD8 T cells . In a model of pulmonary influenza infection, both lymph node resident (CD8α + ) and migratory DCs originating in bronchial epithelium presented class I-restricted antigens to specific CD8 T cells . DCs can exogenously acquire antigen, store it in endocytic compartments without proteolysis, and transfer intact (unprocessed) antigen to B lymphocytes . These observations may lead to DCVs being engineered for migration and antigen transfer rather than direct presentation to T cells.
Dendritic cells can also communicate with each other via secretion of small membrane-bound vesicles (exosomes) derived from the late endocytic compartment. These can contain microRNAs, which are important in the regulation of DC maturation . Immature DCs can release exosomes containing functional peptide–MHC complexes, and this is directly relevant to nucleic acid transfection of DCs . It can be envisaged that even if only a small proportion of the total DC population expressed the antigen, the epitopes (peptide–MHC complexes) could be distributed to the remaining “nontransfected” DCs, leading to amplification of the immune response.
Manipulation of DCV Costimulatory Function
DCV Maturation in Clinical Trials
In vaccine trials, the maturation paradigm requires treating cultured DCVs with stimuli to induce functional DC profiles, characterized by measurable phenotypes, considered desirable for activation of T lymphocyte responses in vivo . These include, first, a switch from antigen acquisition to enhanced processing and presentation with increased surface expression of MHC, particularly stabilization of cell surface peptide–MHC class II. Second, there is an increase in cytokine release, polarized to the stimulation of cytotoxic and IFN-γ-secreting Th1 helper T lymphocyte responses. Central in this is the release of the active p70 IL-12 component and low release of IL-10. Importantly, acquisition of competence to release IL-12 on subsequent cellular interactions, such as CD40 ligation, rather than immediate and transient IL-12 secretion may be the better marker of maturation . Induction of DC-derived IL-15 is important in driving high-affinity CD8 CTL responses . Third is the upregulation of cell surface costimulatory molecules, including CD80, CD83, and CD86. Fourth is the acquisition of a migratory phenotype competent for lymph node homing, particularly expression of CCR7, which is a receptor for ligands CCL19 and CCL2 .
In clinical trials to date, the most widely used method of DCV maturation has been the use of a cocktail of available synthetic cytokines, TNF, IL-6, and IL-1β with PGE 2 . Measurements of DCV maturation markers have generally not been systematically presented in clinical DCV preparations in the majority of trials . Our experience indicated that the expression of these markers is variable between DCV preparations subjected to the same maturation stimuli, but this did not neatly map onto clinical or immune outcome . Both in vitro and in vivo , cytokine matured DCs also promote the expansion of regulatory T lymphocyte numbers through interactions that depend on cell contact mediated by CD80 and CD86 and on DC-sourced IL-2 . IL-6 may be omitted without impairing DCV function . The rationale for including PGE2 is to promote DCV migration via CCR7 upregulation, however in the tumor microenvironment this may favor TH2 rather than TH1 T cell responses and a reduction in IL12 and increased IL10 release by DCVs . PGE 2 treatment of DCs induces expression of indoleamine 2,3 dioxygenase (IDO) and this leads to both tryptophan depletion and expression and secretion of CD25 that acts as an IL2 decoy receptor. Both factors contribute to impaired T cell proliferation in response to PGE 2 -treated DCs . Conversely, vaccination with phenotypically immature DCVs is not necessarily illogical providing that subsequent maturation takes place in vivo .
The evidence that matured DCVs are clinically more effective than immature DCVs (i.e., not treated with a maturation stimulus) is suggestive but very incomplete, hampered greatly by the lack of randomized clinical trials comparing DCV protocols. One human in vivo experiment demonstrated that tolerization induced by DCVs presenting an influenza matrix peptide was attributable to their immature phenotype . In a small cohort of patients with melanoma vaccinated with both immature and mature moDCs presenting different epitopes, it appeared that matured preparations were more immunogenic in vivo . In another trial in melanoma patients, 10 patients receiving THF-α and PGE 2 -matured moDCVs appeared to have stronger delayed-type hypersensitivity cutaneous reactions and responses to KLH than the previous cohort of 9 patients receiving immature DCs . Cytokine and PGE 2 -matured moDCs appear to migrate more effectively from the injection site to draining lymph nodes . Meta-analysis reported odds ratios for clinical benefit favoring matured versus immature DCVs in trials for patients with prostate but not renal cancer . In summary, DCV trials must be interpreted cautiously because even preparations exposed to maturation stimuli in vitro may not be functionally optimal to induce T cell responses and phenotypic markers of maturation may not be good biomarkers typing effective vaccine cells.
Integrated Cell Signaling
Maturation and licensing have to be understood as a process in which sustained, diverse, and sequential discrete signals are integrated over a defined time window. Included in the molecular basis for this is that the key signaling molecule NF-κB is continually degraded and resynthesized, requiring sustained and multiple signals over time. A consequence is that there is a threshold for DC activation reached over a period of time, during which the cell is progressively remodeled so that the requirements for late transcription of genes are met. During this time, regulatory feedback occurs, including degradation of positive signaling components and expression of molecules mediating suppression such as the suppressor of cytokine signaling-1 (SOCS1). The integration of signals within specific DC types can also be understood as combination codes that release distinct patterns of DC function, each of which may include components that might promote or suppress T lymphocyte activation. The complexity of signaling within DCVs impacts on their competence when used as vaccines and serves as a target for ex vivo manipulation going beyond culture in various cocktails of synthetic cytokines. Additional signaling pathways can be recruited that mimic the role of DCs in vivo as sentinels recognizing microbial pathogens. All of these signaling pathways are susceptible to genetic manipulation enhancing or subverting physiological DC function .
Pathogen-Associated Molecular Patterns
Summary of PAMP
TLRs are a family of proteins within varied cellular locations and interacting with different PAMPs. All TLRs signal through the MyG88 adaptor protein and can activate NF-κB. TLR3 and -4 also signal through a different path, resulting in type I IFN release . Other innate systems in DCs for recognition of microbial products include the retinoic acid inducible gene (RIG)-I-like receptors, which can also induce type I IFN. C-lectin receptors also recognize bacterial and fungal carbohydrate, mediating receptor-mediated antigen uptake. The Nod-like receptors also signal through NF-κB or other proinflammatory pathways.
Expression of PAMP on DCs
The expression of TLRs on various DC types has been reviewed . MoDCs, the most common DCV vehicle, particularly express TLR4 and TLR2—receptors for bacterial products LPS and lipoteichoic acid respectively, and the TLRs are located on the plasma membrane. Ligation of either receptor results in pleiotropic events. TLR4 stimulation results in upregulation of surface costimulatory molecules CD80, CD86, and CD83, as well as the lymph node homing receptor CCR7. Cytokine secretion includes IFN-β, IFN-γ, IL-1β, IL-12p70, IL-13, and IP-10. Endocytic function is reduced. TLR2 ligation results in secretion of cytokines IL-6, IL-8, IL-10, IL-12, and TNF-α. TLR7–TLR9, which recognize nucleic acids, are present within endosomes. Expression of TLR7 and TLR9 is uncertain or nonexistent in moDCs. TLR8 is expressed, and stimulation can result in increased secretion of TNF-α, IL-8, and IL-12p40 as well as chemokines MCP-1 and CCL2–CCL5. Circulating mDCs show a TLR receptor expression pattern that overlaps with that of moDCs—notably expressing more TLR3, which recognizes double-stranded RNA, stimulating IFN-α or IFN-β expression. In contrast, pDCs isolated from blood are skewed to expression of TLR7 and TLR9. Stimulation through these upregulates surface costimulatory molecules, CCR7, and cytokines, including IFN-α.
Functional Effects of PAMP Stimulation
In DCV clinical trials, the standard maturation cocktail of proinflammatory cytokines (TNF-α, IL-6, and IL-1β) plus PGE 2 (so-called standard DC) results in mature moDCs based on expression of CD80, CD83, and CD86. However, it is widely reported that standard DCs secrete only limited quantities of IL-12p70 , at least in human serum or serum-free medium , and this has been the major driver of further development of alternative protocols.
Potential TLR ligands available to mature moDCVs include LPS (TLR4), poly I:C (TLR3), and R848 and imiquimod (TLR7/8). TLR9 agonists such as DNA-containing unmethylated CpG oligodeoxynucleotides will not stimulate moDCVs but are relevant for pDCs . In moDCs, R848, poly I:C, and LPS each induce expression of surface costimulatory molecules and secretion of IL-12p70. Furthermore, exploiting signaling through different cellular compartments in maturation cocktails, R848 synergizes with either poly I:C or LPS across a range of concentrations inducing expression of multiple cytokines, notably IL-12p70 and IL-23p19 and the lymph node homing receptor CCR7. R848 plus LPS also skewed the relative expression of Notch ligands driving TH-1 versus TH-2 T cell differentiation. Furthermore, addition of IFN-γ or particularly CD40 signaling to TLR ligand combinations induced a further amplification in response, indicating that moDCs have a high capacity for IL-12p70 induction only deployed by optimal combinatorial signaling . The synergy between stimulation among different TLRs may be partly mediated by a positive feedback loop of type I IFN induction .
It is thought that clinical efficacy requires DCVs to migrate to secondary lymphoid tissue to activate T cell responses. As discussed previously, moDCs stimulated by either or especially both R848 and poly I:C release substantial IL-12p70, and this is further enhanced by addition of IFN-γ or IFN-α. The migration of these TLR DCs, however, is relatively poor, whereas the addition of PGE 2 increases CCR7 expression, random motility, and chemotaxis to CCL22. Addition of PGE 2 to TLR DCs reduces spontaneous IL-12p70 production. Notably, however, secondary IL-12p70 release on contact with T cells or on CD40 ligation was retained in TLR DCs matured in PGE 2 . Protocols combining TLR agonism with PGE 2 and requiring CD40 ligation or other licensing by T cells point the way to optimized mature vaccines that can migrate from vaccine site to lymphoid tissue and interact to drive TH1 differentiation . Interestingly, a comparison of standard moDCs with those matured with IL-1β, TNF-α, IFN-α, IFN-γ, and poly I:C (termed α-DC) confirmed that the latter produced much more IL-12 and were better at stimulating tumor-specific cytotoxic T cells in vitro but had a lower CCR7 expression and responsiveness to CCR-binding chemokines. However, both standard and α-DC showed the similar migration to lymph node in vivo following intradermal injection. The difference between the two DC preparations in cell surface CCR7 levels and in response was accounted for by DC release of CCR7 ligand CCL19, which is inhibited by PGE 2 . It appears that removal from maturation conditions and injection abrogates endogenous CCL19 secretion. Conversely, restimulation with CD40 ligand (CD40L) caused CCL19 secretion to recur in α-DC. This work is limited in scale but may suggest that moDCs activated by TLR ligands without PGE 2 may function better as vaccines. They appear to exhibit migratory capability in vivo despite the contrary findings in vitro . Unlike PGE 2 -treated DCVs, following licensing in the lymph node, they may be more effective in recruiting CCR7 + T cells through CCL19 release . DCs matured with TLR agonists, poly I:C or LPS, cultured with T cells in vitro lead to much less expansion of CD4 + FoxP3 high regulatory T cells .
A further advance is the demonstration that available clinical-grade vaccines against microorganisms can serve as TLR agonists inducing maturation and TH1 polarizing competence in moDCs. Screening of 15 vaccines identified a cocktail of bacille Calmette–Guerin, Influvac, and Typhim with PGE 2 that induced optimal expression of costimulatory surface molecules, CCR7 and IL-12p70 . Of note, an effect of poly I:C stimulation is the induction of an antiviral state (mimicking phagocytosis of certain virus-infected cells ) that antagonizes antigen expression from RNA transfected into moDCs . However, use of the three-vaccine cocktail induced little or no expression of the viral sensor RIG-I and other components of antiviral pathways that might limit antigen expression from transfected RNA, and indeed efficient antigen expression from electroporated RNA was demonstrated .
One interesting question is whether vectors used to introduce antigen genes into DCVs can themselves induce DC maturation. For example, lentiviral transduction of immature moDCs showed a concentration-dependent efficiency of expression of the transgene for which only the higher concentrations resulted in upregulation of costimulatory molecules, MHC, IL-6, IL-8, and TNF-α and low-level secretion of IL-12 similar to standard matured DCs. The effects of lentiviral vectors on DC maturation may vary according to the DC type and source . Coincubation of immature DCVs with RNA was reported to upregulate CD83 and CD86 in a dose-dependent manner. Only immature DCVs take up RNA in this manner. Although still phenotypically immature on injection, it remains possible that RNA transfection committed the DCVs to maturation completed in vivo . The key mechanisms potentially involved in upregulation of DC maturation by viruses include sensing of single-stranded viral RNA by RIG-I and detection of double-stranded viral RNA by TLR3 .
TLR signaling is being directly linked to antigen loading in DCs through several approaches to bring about both DC maturation and epitope presentation. Engineered fusion proteins comprising proteins marking tissue damage as TLR agonists linked to tumor antigen are being developed. Although reported as methods of direct vaccination with the construct, these may also operate in a DC vaccination system . Another promising approach described is cotransfection of DCs with RNA encoding tumor antigen Melan-A alongside constitutively active TLR4 . The insertion through electroporation of poly I:C into tumor cells triggers RIG-like receptor pathways, leading to autophagy and apoptosis. Such cells can then trigger maturation in cultured DCs .
Manipulation of Outputs of Maturation
One approach to DC maturation has been to directly express T cell stimulatory factors through gene transfer as an adjunct to maturation. For example, the use of a fowlpox vector to transduce human moDCs, DCs with costimulatory CD80, and adhesion molecules LFA3 and ICAM-1 (termed TRICOM) increased expression of these molecules without altering other markers of maturation and enhanced T cell stimulatory properties. Transduction of DCs with TRICOM and genes encoding tumor antigens results in all transgenes being expressed, and in CD40L-matured moDCs, such an approach enhanced both the avidity for target epitope and cytokine profile of responding T cells. Vaccination of people with cancer with autologous immature moDCVs transduced with fowlpox encoding CD80, LFA3, ICAM-1, and CEA resulted in a probable increase in CEA responders in 10 of 14 patients.
Maturation protocols can result in IL-10 release, potentially undermining the desired TH1 skewing of the response to DCVs. The transfection of DC tumor fusion cells with both poly I:C and a small inhibitory RNA for IL-10 generated cells that elicited an efficient TH1 tumor-directed response in vitro .
Licensing through Interaction with Other Cells
The paradigm for DC activation includes the important role of licensing—that is, further functional change resulting from interactions with other cell types predominantly taking place within lymph nodes. Mimicking licensing in vitro or engineering its occurrence in vivo is a key advancement in DCV development. For example, it is recognized that exposure to IFN-γ at the time of TLR4 ligation contributes to DC activation and polarization of function. The release of IL-12p70 has been described as requiring the sequential and coordinate exposure to IFN-γ, PAMP, and CD40 ligation. Furthermore, the order in which the stimuli are received affects outcome in that optimal IL-12p70 release results from IFN-γ used before TLR exposure and TLR3 or -4 agonists used before TLR8.
CD40L is a member of the TNF superfamily, with expression upregulated early during activation of CD4 + T lymphocytes and expressed at lower levels on other inflammatory cell types. CD40 expression is upregulated on DCs through maturation, and its ligation induces further changes, such as expression of costimulatory ligands for T cell activation, OX40L and 4-1BBL. CD40 stimulation also induces increased and more durable IL-12 secretion as well as anti-apoptotic pathways. At least in murine models, CD40L is also expressed on DCs, upregulated by TLR agonism, and contributes to CD4 + T cell-independent immune responses. In a murine model, DC–tumor fusion cells were transduced with an adenovirus expressing CD40L, resulting in enhanced expression of T cell costimulatory molecules and MHC class II and providing evidence of increased efficacy in vivo as an antitumor vaccine. A number of studies report engineering the CD40 system in DCVs to enhance licensing. Temporal control of CD40-induced activation has been sought through adenoviral transduction into immature moDCs of an inducible iCD40 gene encoding the CD40 cytoplasmic signaling domain linked to a membrane targeting sequence and a synthetic moiety mediating drug-induced dimerization. Induced iCD40 dimerization upregulated maturation markers CD80, CD83, and CD86 and showed additive effects with TLR4 agonism. The TLR4 and iCD40 combined signaling resulted in higher and longer IL-12 secretion and reduced negative feedback signaling though SOCS1. This approach contributed to TH1 polarization and induction of tumor antigen-specific responses in vitro and in a murine model. Although being developed primarily as novel protein subunit vaccines against infectious disease, constructs linking antigen to CD40L are being investigated as a mechanism for inducing antigen uptake into DCs with licensing. In order to more efficiently direct antigen expression in DCVs and combine this with licensing, an adenoviral transduction has been carried out with an engineered murine or human adaptor molecule, CFm40L or CFh40L, respectively, comprising a cocksackie–adenovirus receptor fused to CD40L. Immature moDCs, transduced with adenovirus encoding viral or tumor antigen, exposed to IFN-γ and treated with the adaptor exhibited enhanced costimulatory properties and induced greater T cell response and TH1 polarization. Similarly, CD40L has been exploited as a mechanism for delivery to DCs of payload in synthetic nanoparticles. These approaches lend themselves to DC-directed in vivo vaccination strategies as well as antigen loading and licensing of DCVs in vitro .
Another example of using nucleic acid transfection to enhance maturation and antigen-presenting capability of the vaccine DCs was described by Bonehill et al. . The target antigen for vaccination was expressed after mRNA electroporation alongside a mix of RNAs encoding CD40L, CD70, and constitutively active TLR4. The result of expression of these molecules was the initiation of a maturation program because CD70 is a costimulatory receptor binding to CD27 and CD40 ligation provides T cell-derived maturation signals to the DCs. There was no loss of transfection efficiency using four RNA species compared to three, and even without an exogenous cytokine stimulus, the vaccine cells secreted IL-12p70 and displayed robust HLA class II and CD83 expression. Melanoma patients receiving TriMix DC vaccination showed expansion of CD8 T cell responses to the vaccinated antigens. On an individual patient basis, use of TriMix DC expressing four melanoma antigens linked to a LAMP-1 targeting sequence via intravenous and intradermal routes led to development of a broad range of CD4 and CD8 T cell responses of various specificities and also a clinical response with reduced metabolic activity of lung, lymph node, and bone metastases.
DC Survival
One concern about the potential immunogenicity of terminally differentiated DC vaccines has been their short life span after administration, which is considered to hinder long-lived contacts between the DCs and T cells that are likely of very low frequency. Two strategies involving genetic modification of DCs have been applied to prolong DC survival: use of small interfering RNA to reduce expression of pro-apoptotic proteins and overexpression of anti-apoptotic factors. In a murine model of vaccination against tumor challenge, Kim et al. . demonstrated a 3- to 10-fold increase in CD8 T cells specific for an HPV E7 epitope if vaccine DCs were pretreated with siRNA against proteins such as BAX and caspase-8. This was associated with prolonged DC survival and also more robust protection from subsequent challenge with an E7-expressing tumor. Similar findings were obtained in a murine model using DCs coexpressing a tumor-associated antigen and the anti-apoptotic protein Bcl-xl.
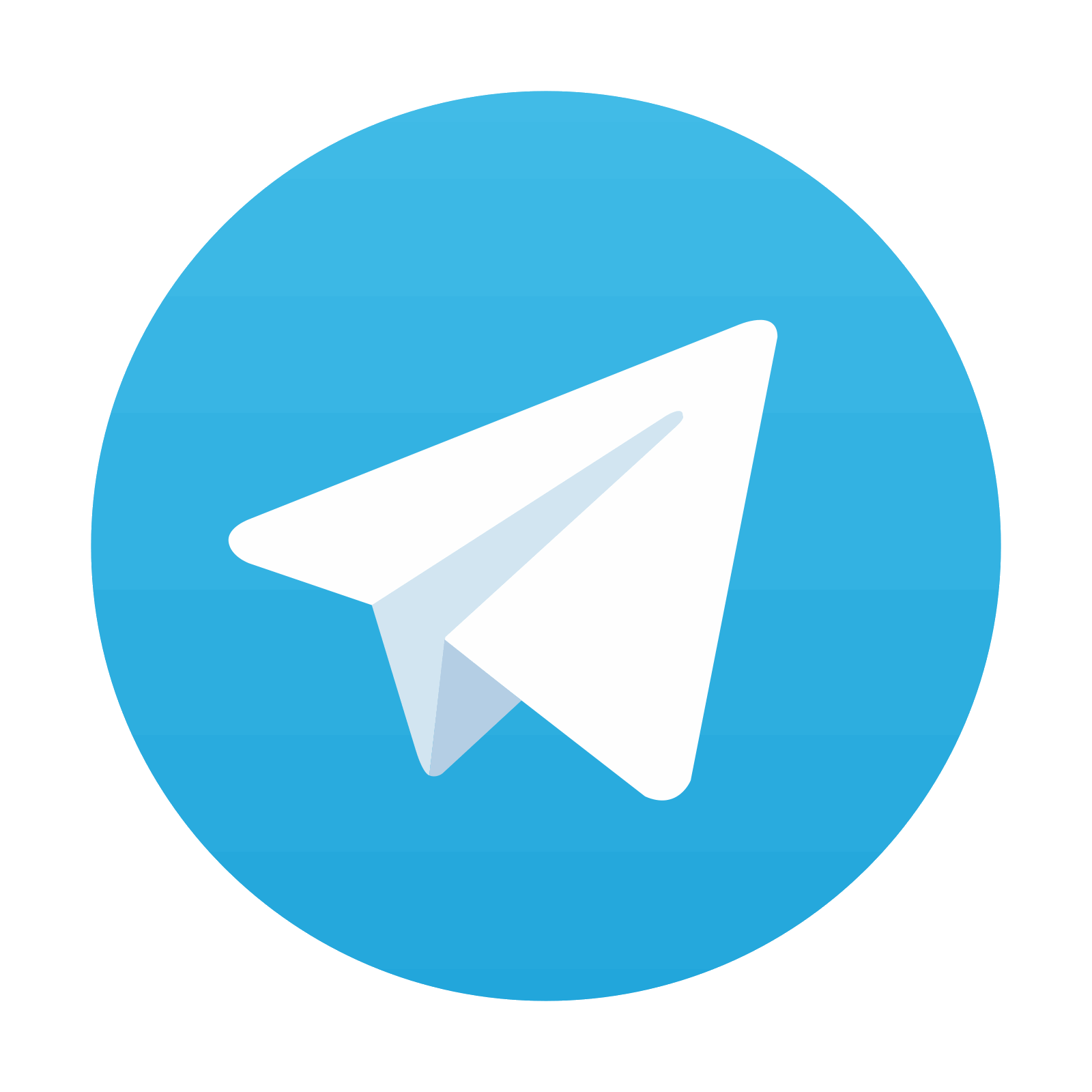
Stay updated, free articles. Join our Telegram channel
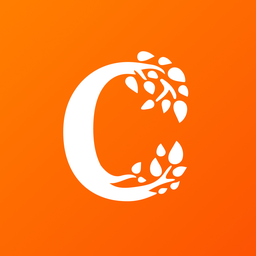
Full access? Get Clinical Tree
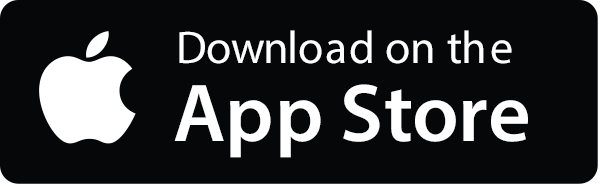
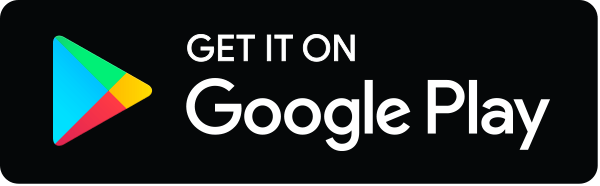