Nicholas A. Kefalides *, Zahra Ziaie, Edward J. Macarak
Connective Tissues and Aging
Aging is a continuous process that constitutes a cycle studded with events that affect all systems in the body, including the connective tissues. The interrelationship between the aging process and connective tissues is complex, involving a variety of factors and interactions acting in a reciprocal fashion. One could inquire into the effects of aging on connective tissues and, conversely, one may ask how the components of connective tissue contribute to the aging process. To answer these questions, it is important to have some understanding of the structural biochemistry of connective tissues, knowledge of the processes involved in their biosynthesis, modification, extracellular organization, molecular genetics, and of the factors affecting the properties of connective tissue cells and the extracellular matrix (ECM). Since the last edition, new data have become available that highlight the progress made regarding the mechanisms responsible for the alterations in connective tissue components in diseases associated with aging. Armed with this knowledge, it becomes apparent that there can be a huge number of events in the development of connective tissues that may be associated, directly or indirectly, with the processes or effects of aging. These have been and continue to be areas of intensive research.
This chapter presents an abbreviated discussion of the various components of the ECM and their structure, molecular organization, biosynthesis, modification, turnover, and molecular genetics. It discusses some concepts on the effects of aging on the ECM and effects of aging on the properties of various connective tissues, as well as the involvement of connective tissue physiology on diseases associated with aging.
Properties of Connective Tissues
The properties of connective tissues are derived primarily from the properties of the components of the ECM surrounding, and secreted by, the cells of those tissues. Some connective tissues, such as cartilage or tendons, are products primarily of a single cell type (e.g., chondrocytes, fibroblasts) whose synthesis and secretion of ECM and other factors largely determine the properties of the tissue. Some structures, such as bone, blood vessels, and skin contain a number of different connective tissue cell types, such as osteoblasts and osteoclasts in bone, endothelial, and smooth muscle cells, fibroblasts in blood vessels, and fibroblasts, epithelial cells, and adipocytes cells in skin, which contribute to their structural and functional properties. Other tissues and organs, such as cardiac muscle and kidney, may have properties dependent on connective tissue components whose biologic roles are separate from the major physiologic function of the tissue and that may influence the properties of that tissue during the process of aging. Different cell types will exhibit different phenotypic patterns of ECM production that in turn will influence the structural properties of a given connective tissue.
The major components of the ECM fall into three general classes of molecules: (1) the structural proteins, which include the collagens (of which there are now 28 recognized types) and elastin; (2) the proteoglycans, which contain several structurally distinct molecular classes, such as heparan sulfate and dermatan sulfate; and (3) the structural glycoproteins, exemplified by fibronectin (FN) and laminin (LM), whose contributions to the properties of connective tissues have been recognized only within the past 35 to 40 years. The interactions among these materials determine the development and properties of the connective tissues.
Collagens
Structure
The collagens are a family of connective tissue proteins characterized by the presence of three polypeptides called alpha chains, which contain molecular domains that are wound together in a ropelike super helix. Collagens are rich in the amino acids proline and glycine, which play roles in the formation and stability of the triple-stranded super helix. The reader is referred to two reviews on collagen biochemistry.1,2
The genes of at least 28 distinct collagen types have been characterized.3 The interstitial collagens, types I, II, III, and V, exist as large extended molecules that tend to organize into fibrils that may be heterotypic1—that is, there may be more than one collagen type within these fibrils.4 Type IV collagen, also termed basement membrane (BM) collagen, does not exist in fibrillar form but is in a complex network of collagen molecules linked by disulfide and other cross-linkages and associated with noncollagenous molecules, such as LM, entactin, and proteoglycans, to form an amorphous matrix.5,6 Although at least 28 collagen types are recognized, the protein of only the first 11 collagens has been isolated from tissues.
Table 19-1 presents a summary of the collagen family (a modification of the one reported by Canty and Kadler3). There are 46 genes corresponding to the alpha chains of 28 collagen types. Collagen type I is the most abundant collagen and protein in the body. The basic unit of the type I collagen fibril is a triple helical heterotrimer, tropocollagen, consisting of two identical chains, alpha 1(I), and a third chain, alpha 2(I).1 The other collagen types have been given similar designations; however, some of the types are homotrimers containing three identical chains and some contain three genetically distinct chains.
TABLE 19-1
Collagen Types
Type | Genes | Tissue Distribution |
I | COL1A1, COL1A2 | Skin, tendon, bone, cornea, blood vessels |
II | COL2A1 | Cartilage, intervertebral discs, vitreous body |
III | COL3A1 | Skin, blood vessels |
IV | COL4A1, COL4A2, COL4A3 COL4A4, COL4A5, COL4A6 | Basement membranes (BMs) |
V | COL5A, COL5A2, COL5A3 | Placenta, skin, cardiovascular system |
VI | COL6A1, COL6A2, COL6A3 COL6A4, COL6A5, COL6A6 | Cornea, blood vessels, lung, testis, colon, kidney, liver, spleen, thymus, heart, skeletal muscle, articular cartilage |
VII | COL7A1 | Skin, cornea, gastrointestinal tract |
VIII | COL8A1, COL8A2 | Cardiovascular system, placenta, cornea |
IX | COL9A1, COL9A2, COL9A3 | Cartilage, cornea |
X | COL10A1 | Cartilage |
XI | COL11A1, COL11A2, COL2A1 | Cartilage |
XII | COL12A1 | Tendons, periosteum |
XIII | COL13A1 | Many tissues |
XIV | COL14A1 | Skin, bone, cornea, blood vessels |
XV | COL15A1 | Placenta, heart, colon |
XVI | COL16A1 | Placenta, heart, colon |
XVII | COL17A1 | Skin hemidesmosomes |
XVIII | COL18A1 | Several tissues, particularly kidney and liver |
XIX | COL19A1 | Rhabdomyosarcoma cells |
XX | COL20A1 | Corneal epithelium, embryonic skin, sternal cartilage, tendon |
XXI | COL21A1 | Heart, stomach, kidney, skeletal muscle, placenta, blood vessel |
XXII | COL22A1 | Articular cartilage, skin, tissue junctions—cartilage synovial fluid, myotendinous junctions in skeletal and heart muscle |
XXIII | COL23A1 | Lung, cornea, tendon, brain, skin, kidney |
XXIV | COL24A1 | Bone and cornea |
XXV | COL25A1 | Amyloid plaques in the brain |
XXVI | COL26A1 | Testis, ovary |
XXVII | COL27A1 | Cartilage, tendon, stomach, lung, gonad, skin, cochlea, tooth |
XXVIII | COL28A1 | Kidney, skin, calvaria, nerves, BM of certain Schwann cells |
The collagen alpha chain has a unique amino acid composition, with glycine occupying every third position in the sequence. Thus, the collagenous domains consist of a repeating peptide triplet, -Gly-X-Y-, in which X and Y are amino acids other than glycine. A large percentage of amino acids in the Y position is occupied by proline. In addition, collagen contains two unique amino acids derived from posttranslational modifications of the protein, 4- and 3-hydroxyproline and hydroxylysine. The presence of 4-hydroxyproline provides additional sites along the alpha chain capable of forming hydrogen bonds with adjacent alpha chains, which are important in stabilizing the triple helix so that it maintains its structure at body temperatures. If hydroxyproline formation is inhibited, the triple helix dissociates into its component alpha chains at 37° C, making it structurally unstable.
The presence of glycine in every third position, along with the extensive hydrogen bonding, provides the triple helix with a compact protected structure resistant to the action of most proteases. The alpha chains of the collagen superfamily are encoded with information that specifies self-assembly into fibrils, microfibrils, and networks that have diverse functions in the ECM.6 The structures of collagens can be stabilized further through the formation of covalent cross-linkages derived from modification and condensation of certain lysine and hydroxylysine residues on adjacent alpha chains.2 Cross-linkage formation is important in stabilizing collagen fibrils and contributes to their high tensile strength, equivalent to that of fine steel wire.
Biosynthesis
Type I collagen alpha chains are synthesized as a larger precursor, procollagen, containing noncollagenous sequences at their C and N termini.7 As each pro–alpha chain is synthesized, intracellular prolyl and lysyl hydroxylases act to form hydroxyproline and hydroxylysine. The triple helix is formed intracellularly and stabilized by the formation of interchain disulfide bonds near the carboxyl termini of the component pro–alpha chains. After secretion of the triple helical collagen, procollagen peptidases remove most of the noncollagenous portions at each end of the procollagen. Extracellular lysine and hydroxylysine oxidases oxidize the epsilon amino groups of lysine or hydroxylysine to form aldehyde derivatives, which can go on to form Schiff base adducts, the first cross-linkages. These can rearrange and become reduced to form the various other cross-linkages. Increased number of collagen cross-linkages have been reported in a pathologic state known as scleroderma.
Degradation of Connective Tissue Components
The role played by matrix metalloproteinases (MMPs) in connective tissue turnover has gained prominence in the past 40 years as information on the mechanisms whereby MMPs mediated synovial joint inflammation, as well as ECM turnover, in arthritides became available.8 Extracellular degradation of collagen is accomplished by enzymes known as tissue collagenases. These enzymes cleave triple helical collagen at a site three quarters from the amino terminus, resulting in the formation of two triple helical fragments that become denatured at temperatures above 32° C to form nonhelical peptides, which can be degraded by tissue proteinases. Cleavage by tissue collagenase is considered to be the rate-limiting step in the collagenolysis of triple helical collagen. Collagenolysis is the subject of reviews by Kleiner and Stetler-Stevenson9 and Tayebjee and colleagues.10
Collagenolysis is an important physiologic process responsible to a large extent for the repair of wounds and processes of tissue remodeling in which undesired accumulations are removed as new connective tissue is laid down. However, in conditions such as rheumatoid arthritis and osteoporosis (OS), as well as aging, the production of collagenases may be stimulated, resulting in an elevated degradation of synovial tissue or bone. Degradation of elastin by elastases, belonging to a family of serine, metallo, or cysteine proteinases, gives rise to the generation of elastin fragments, designated as elastokines.11
Tissue collagenases are secreted by connective tissue cells as a precursor procollagenase, which must be activated to become enzymatically functional. This can be achieved in vitro by the action of trypsin on the latent enzyme. Other proteinases, including lysosomal cathepsin B, plasmin, mast cell proteinase, and plasma kallikrein, also can activate latent collagenases. Thus, inflammatory cells can secrete factors that lead to collagenase activation, accounting for the inflammatory sequelae of the arthritides. Collagenases are also under the influence of plasma inhibitors, of which α2-macroglobulin accounts for most of the inhibitory process. In addition, inhibitors of plasminogen activation can indirectly prevent the activation of procollagenases by plasmin. Fibroblasts and other connective tissue cells also secrete inhibitors of collagenases, suggesting a complex system of extracellular control of collagenolysis.9,10
Elastin
The biochemistry and molecular biology of elastin have been subjects of excellent reviews.12,13 As in interstitial collagens, glycine makes up about one third of the amino acid content of elastin. Unlike collagen, however, glycine is not present in every third position. In addition, elastin is an exceedingly hydrophobic protein, with a large content of valine, leucine, and isoleucine.
Elastin is synthesized as a precursor molecule, tropoelastin, with a molecular weight of about 70 kDa. However, in tissues, elastin is found as an amorphous macromolecular network. This is because of the condensation of tropoelastin molecules through the formation of covalent cross-linkages unique to elastin. These cross-linkages arise through the condensation of four lysine residues on different tropoelastin molecules to form the cross-linking amino acids, desmosine and isodesmosine, that are characteristic of tissue elastin. The reader is referred to reviews by Bailey and associates2 and Wagenseil and Mecham12 for a more detailed discussion of collagen and elastin cross-linking.
The hydrophobicity, together with the formation of cross-linkages, endow elastin with its elastic properties as well as its extreme insolubility and amorphous structure. Elastin accounts for most of the elastic properties of skin, arteries, ligaments, and the lungs. The presence of elastin has been demonstrated in other organs, such as the eye and kidney. In most tissues, elastin is found in association with microfibrils, which contain several glycoproteins, including fibrillin. Microfibrils have been identified in many tissues and organs, and the importance of their assembly as determinants of connective tissue architecture has been brought into focus by the identification of mutations in fibrillin in the heritable connective tissue disorder, Marfan syndrome.13
An elegant review has summarized knowledge of the structure of the elastin gene, including consideration of the heterogeneity observed in immature mRNA due to alternative splicing in the primary transcript.14 Analyses of the bovine and human elastin genes have revealed the separation of those exons coding for distinct hydrophobic and cross-linking domains. Comparison of the cDNA and genomic sequences, as well as S1 analyses, have demonstrated that the primary transcript of both species is subject to considerable alternative splicing. It is likely that this accounts for the presence of multiple tropoelastins found in several species. It has been suggested that the differences in alternative splicing may be correlated with aging.14
Proteoglycans
Proteoglycans are characterized by the presence of highly negatively charged, polymeric chains (glycosaminoglycans [GAGs]) of repeating disaccharide units covalently attached to a core protein. The disaccharide units comprise an N-conjugated amino sugar, either glucosamine or galactosamine, and a uronic acid, usually D-glucuronic acid or, in the case of dermatan sulfate, heparan sulfate, and heparin, L-iduronic acid. In cartilage and in the cornea, another GAG, keratan sulfate, containing D-glucose instead of a uronic acid, has been demonstrated. The amino group of the hexosamine component is generally acetylated, and the GAGs are usually O-sulfated in hexosamine residues with some N-sulfation, instead of acetylation, in the case of heparan sulfate and heparin. Depending on the source and type of proteoglycan, the number of GAGs attached to the core protein can vary from three or four to more than 20, with each GAG having a molecular size in the tens of thousands of daltons. In addition, as in the case of the cartilage proteoglycans, there may be more than one type of GAG attached to the core protein. In cartilage, several proteoglycan molecules may be associated with another very large GAG, hyaluronic acid, consisting of disaccharide units of glucuronyl N-acetylglucosamine. The compositional structure of the GAGs is summarized in Table 19-2.
TABLE 19-2
Properties and Tissue Distribution of Glycosaminoglycans (GAGs)
GAGs | Composition | Tissue Distribution |
Hyaluronic acid | N-Acetylglucosamine D-Glucuronic acid | Blood vessels, heart, synovial fluid, umbilical cord, vitreous |
Chondroitin sulfate | N-Acetylgalactosamine D-Glucuronic acid 4- or 6-O-sulfate | Cartilage, cornea, tendon, heart valves, skin |
Dermatan sulfate | N-Acetygalactosamine L-Iduronic acid 4- or 6-O-sulfate | Skin, lungs, cartilage |
Keratan sulfate | N-Acetyglucosamine D-Galactose O-Sulfate | Cornea, cartilage, nucleus pulposus |
Heparan sulfate | N-Acetylglucosamine | Blood vessels, basement membranes, lung, spleen, kidney |
Heparin | N-Sulfaminoglucosamine D-Glucuronic acid L-Iduronic acid O-Sulfates | Mast cells, lung, Glisson membranes |
The overall effect of these structures is the creation of huge, negatively charged highly hydrophobic complexes. The hydration and charge properties of these complexes cause them to become highly extended, occupying a hydrodynamic volume in the tissue much larger than that which would be predicted from their chemical composition. In the case of synovial cartilage, it is suggested that the hydration endows the tissue with shock-absorbing properties in which applied pressure to the joint is counteracted by the extrusion of water from the complex, forcing a compression of the negative charges within the molecule. On the release of pressure, the electronegative repulsive forces drive the charges apart, with a concomitant influx of water to restore the initial hydrated state. The metachromatic staining properties of connective tissues are mainly because of their proteoglycan content. There have been several excellent reviews of proteoglycan biochemistry.15–17
In recent years, several proteoglycans have been identified in the pericellular environment, associated with cell surfaces or interacting with ECM components, such as interstitial collagens, FN, and transforming growth factor-β (TGF-β). Reviews by Groffen and coworkers15 and Schaefer and Iozzo16,17 have described the structures of the protein cores and their gene organization, functional characteristics, and tissue distribution. Table 19-3 (a modification of that published by Schaefer and Iozzo16) lists the biologic characteristics of pericellular proteoglycans. Several of the proteoglycans on the list constitute a group of small, leucine-rich proteoglycans (SLRPs). Notable among them are decorin17 and perlecan.18 They are multidomain assemblies of protein motifs with relatively elongated and highly glycosylated structures and have several protein domains shared with other proteins. In their review, Groffen and colleagues15 discussed the role of perlecan as a crucial determinant of glomerular BM permselectivity and suggested that the additional presence of agrin, another heparan sulfate proteoglycan species, makes the latter important contributors to glomerular function.
Lumican, one of the leucine-rich proteoglycans, is found in relative abundance in articular cartilage,17 which, along with its size, varies with age. In adult cartilage extracts, it exhibits a molecular size in the range of 55 to 80 kDa. Extracts from juvenile cartilage have a more restricted size variation corresponding to the higher molecular size range present in the adult. In the neonate, the sizes are in the range of 70 to 80 kDa.
The biosynthesis of proteoglycans begins with the synthesis of the core protein. The sugars of the GAG chain, in most cases, are sequentially added to serine residues of the protein using uridine diphosphate conjugates of the component sugars, with sulfation following as the chain elongates. Most of the chain elongation and sulfation is associated with the Golgi apparatus. The degradation of proteoglycans is mediated through the action of lysosomal glycosidases and sulfatases specific for the hydrolysis of the various structural sites within the GAG chain. Genetic abnormalities in the production or synthesis of these enzymes have been shown to be the main causes of the mucopolysaccharidoses, whose victims may exhibit severe tissue abnormalities and a high incidence of mental retardation.
Structural Glycoproteins
In addition to the collagen and elastin components of connective tissues, there are groups of glycoproteins, the structural glycoproteins, that have important roles in the physiology and structural properties of connective and other types of tissues. These proteins, which include FN, LM, entactin-nidogen, thrombospondin (TSP), and others, are involved during development, in cell attachment and spreading, and in tissue growth and turnover.
Fibronectin
One of the best characterized of the structural glycoproteins is fibronectin. It was originally isolated from serum, where it was referred to as cold-insoluble globulin (CIG). As it became recognized that FN was an important secretory product of fibroblasts and other types of cells, and was involved in cell adhesion, the term fibronectin replaced CIG. Comprehensive reviews on the structure and function of FN have been published by Haranuga and Yamada20 and Schwarzbauer and DeSimone.21
FN exists as a disulfide-linked dimer with a molecular weight of about 450 kDa, with each monomer having a molecular size of 250 kDa. FN exists in at least two forms, a cell-associated form and a plasma form. Plasma FN is synthesized by hepatocytes and secreted into the circulation. It is somewhat smaller and more soluble at a physiologic pH than the cellular form. Spectrophotometric and ultracentrifugal studies have indicated that both forms are elongated molecules composed of structured domains separated by flexible, extensible regions. Limited proteolytic digestion studies have revealed the presence of specific binding sites for a number of ligands, including collagen, fibrin, cell surfaces, heparin (heparan sulfate proteoglycan), factor XIIIa, and actin.
FN plays a role in blood clotting by becoming cross-linked to fibrin through the action of factor XIIIa transamidase, which catalyzes the final step in the clotting cascade.22 Fibroblasts and other cell types involved in the repair of injury adhere to the clot by interacting with the cell-binding domain of FN. FN contains a unique peptide sequence, arginyl-glycyl-aspartyl-serine (RGDS, RGD), which binds to specific cell surface proteins (integrins) that span the plasma membrane.21 Purified RGD can inhibit FN from binding the cells and can even displace bound FN. The integrins have a complex molecular organization and appear to interact with certain intracellular proteins, thereby providing a mechanism for the control of a number of events by components of the extracellular environment.
FN is encoded by a single gene, and its complete primary structure has been determined by the DNA sequencing of overlapping complementary DNA (cDNA) clones.23 From such studies, it became recognized that there are peptide segments derived from alternative splicing of FN mRNA at three distinct regions, termed extradomain A (ED-A), ED-B, and connecting segment (CS) III. A middle region of FN containing homologous repeating segments of about 90 amino acids, called type III homologies, has been identified.24,25 Using immunologic techniques with monoclonal antibodies, it was shown that the ED-A exon is omitted during splicing of the FN mRNA precursor in arterial medial cells; the expression of FN containing ED-A, however, is characteristic of multiple cell types involved in wound healing and tissue and organ fibrotic diseases characterized by the overproduction of connective tissue proteins. In such disorders, EDA-FN synthesis precedes that of collagens and is a requirement for the TGF-β–induced differentiation of fibroblasts into myofibroblasts. The contributions of myofibroblast differentiation and expression of the EDA-FN isoform to the aging process are problematic because of their close association with the early stages of fibrotic diseases.26 Genetic studies have shown that ED-A is not required for normal development, but significant abnormalities were noted in adult mice that lacked the ED-A gene.27 Increased ED-A FN has been demonstrated in the skin of patients with scleroderma.28 ED-A FN also is found during embryonic development where it plays a role in cell migration. In addition, recent evidence has shown the presence of EDA-FN in keloid scars.29
This could be the source of differences between the plasma and cellular forms of FN. This phenomenon of alternative splicing may also be involved in the synthesis of collagens and elastin and may well be implicated in the processes of aging.
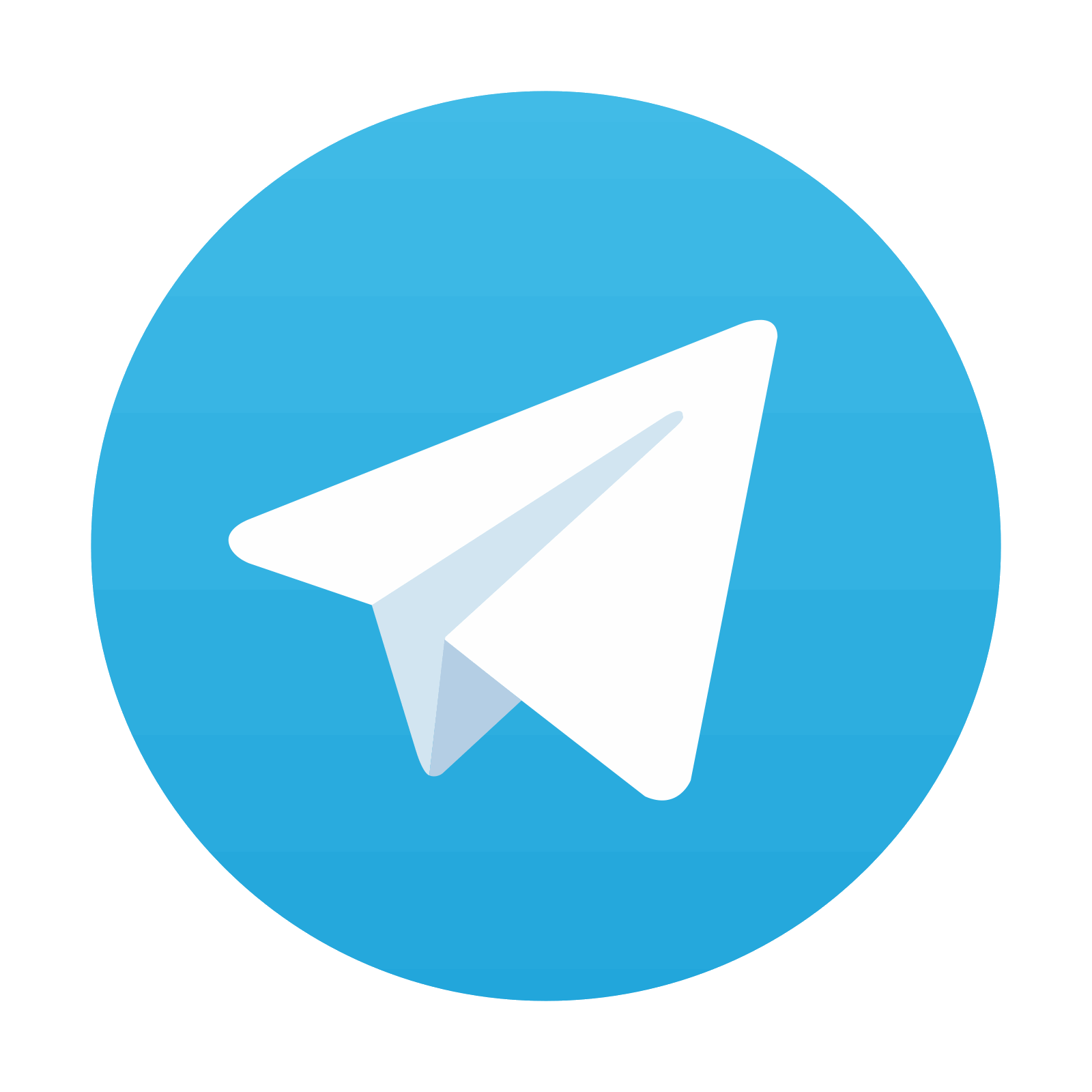
Stay updated, free articles. Join our Telegram channel
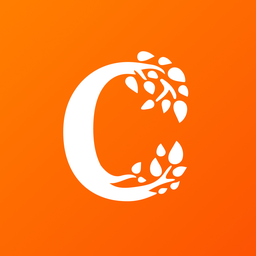
Full access? Get Clinical Tree
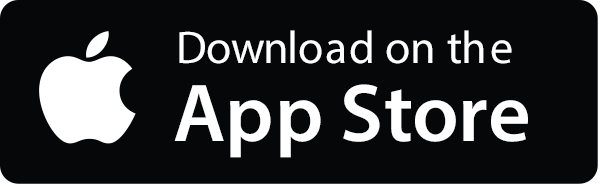
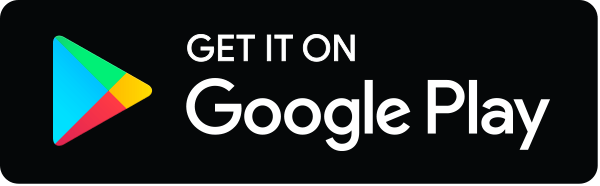