21 Central Nervous System Tumors
Primary central nervous system (CNS) tumors accounted for an estimated 21,810 new cases diagnosed in the year 2008 in the United States,1 and for an estimated 13,810 deaths for the same year. The incidence of brain tumors has been slowly increasing at an average rate of 1.1% per year.2 However, the death rate has been decreasing over time, and the 5-year relative survival rate for primary brain tumors has improved from 24% during 1975 through 1977 to 35% during 1996 through 2003.1 There is an early peak of 2 to 3 per 100,000 in the annual age-specific incidence of primary brain tumors in children up to the age of 4 years. A decline occurs between 15 and 24 years, followed by a steady rise, reaching a plateau of 21 per 100,000 between 75 and 79 years of age.3 Several histopathologically different tumors arise in the brain, reflecting the diversity of phenotypically distinct cells within the CNS, that have a capacity for neoplastic transformation.4 Malignant gliomas are considered first in this chapter. Many of the principles of brain tumor management are discussed in this section. Tumors that are most common in children but that are also seen in adult patients are reviewed in Chapter 54 and are only briefly presented here. The chapter closes with a discussion on the management of metastatic brain tumors, which constitute the most common type of brain tumor encountered.
Malignant Glioma
Epidemiologic Statistics
Malignant gliomas make up 35% to 45% of primary brain tumors, and of these, nearly 85% are glioblastoma multiforme (GBM).4 The incidence of anaplastic astrocytoma (AA) peaks in children younger than 10 years of age and then remains constant in each subsequent decade of life. In contrast, GBM is uncommon before the age of 20 years, whereas the rate of occurrence increases dramatically after the age of 40.3 The incidence of malignant gliomas has increased at least twofold among the elderly during the previous two decades.5
Current knowledge of the molecular biology and genetics of the astrocytic neoplasms has been well summarized.6 Two genetic pathways, a progression pathway and a de novo pathway, that lead to GBM development have been described (Fig. 21-1). Although primary and secondary GBMs are histologically indistinguishable, it is well accepted that distinct molecular aberrations and pathways lead to the formation of primary (de novo) versus secondary GBMs. Primary GBMs are thought to arise in a de novo manner, without any malignant precursor lesion. Amplification of the epidermal growth factor receptor (EGFR) is thought to be a critical event in the molecular cause of primary GBMs. Secondary GBMs, on the other hand, are thought to arise as a result of the malignant transformation of lower grade gliomas, with overexpression of platelet-derived growth factor, fibroblast growth factor–2, and cyclin-dependent kinase 4, as well as p53 mutations and loss of Rb playing major roles in such transformations. Loss of PTEN has been implicated in both pathways, although it is much more common in the pathogenesis of primary GBM.
Several hereditary syndromes are associated with an increased risk of brain tumors (Fig. 21-2). Neurofibromatosis-1 is the most common familial tumor syndrome associated with brain tumors.7 With the exception of Turcot syndrome, all are inherited in an autosomal dominant pattern. In addition to the association between hereditary syndromes and brain tumors, certain families of brain tumor patients have aggregations of brain tumors and extraneural malignancies. Germ-line p53 mutations have been frequently identified in glioma patients with multifocal lesions, a different second primary malignancy, and a family history of cancer.
Although some environmental factors have been linked with brain tumor development, they do not appear to be responsible for most brain tumors.8 Apart from a predisposing genetic syndrome that is present in less than 5% of patients with brain tumors, the only other firmly established cause is ionizing radiation. Radiation-induced gliomas have been reported, and 25% of such cases have arisen in children with acute lymphoblastic leukemia who received prophylactic cranial irradiation and chemotherapy.
Anatomy
The brain is divided into supratentorial and infratentorial compartments by the tentorium cerebelli. The supratentorial compartment includes the cerebral hemispheres and the sellar, pineal, and diencephalic regions, whereas the infratentorial compartment includes the midbrain, pons, medulla, and cerebellum. The cerebral hemispheres are connected by the corpus callosum and are divided into the frontal, parietal, occipital, and temporal lobes. The frontal lobes are concerned with behavior organization, planning and association, and speech; the parietal lobes with motor, sensory, and complex intellectual functions; the occipital lobes with vision; and the temporal lobes with behavior, memory, speech, emotion, and auditory-visual pathways.9 Most malignant gliomas arise in the cerebral hemispheres, and the lobar distribution is directly related to the amount of white matter present in each region.
The brain is housed within the bony calvarium, made up of the frontal, ethmoid, parietal, sphenoid, temporal, and occipital bones. The posterior margin of the lesser wings of the sphenoid marks the anterior aspect of the lateral cerebral (Sylvian) fissure, the junction of the frontal and temporal lobes of the brain. The base of the skull can be approximated on the surface of the patient by a line, called the orbitomeatal or Reid baseline, which extends from the infraorbital rim to the external auditory meatus.9
Pathologic Conditions
Neuropathologists have not agreed on a uniform classification system for brain tumors. However, the fourth edition of the World Health Organization (WHO) classification of tumors of the CNS, published in 2007, is based on the consensus of an international working group of pathologists and geneticists.10 It is the standard for the definition of brain tumors for clinical oncology and cancer research communities worldwide. According to this classification, CNS tumors are classified as follows: (1) tumors of neuroepithelial tissue, (2) germ cell tumors, (3) tumors of cranial and paraspinal nerves, (4) tumors of the sellar region, (5) tumors of the meninges, (6) lymphomas and hemopoietic neoplasms, and (7) metastatic tumors (Table 21-1).
Table 21-1 World Health Organization Classification of Primary Central Nervous System Tumors
Major Classification of Tumors | WHO Grade |
---|---|
Astrocytic Tumors | |
Pilocytic astrocytoma | I |
Diffuse astrocytoma | II |
Anaplastic astrocytomas | III |
Glioblastoma | IV |
Gliosarcoma | IV |
Oligodendroglial Tumors | |
Oligodendroglioma | II |
Anaplastic oligodendroglioma | III |
Oligoastrocytic Tumors | |
Oligoastrocytoma | II |
Anaplastic oligoastrocytoma | III |
Ependymal Tumors | |
Ependymoma | II |
Anaplastic ependymoma | III |
Choroid Plexus Tumors | |
Choroid plexus papilloma | II |
Atypical choroid plexus papilloma | III |
Tumours of the pineal region | |
Pineocytoma | I |
Pineoblastoma | IV |
Neuronal and Mixed Neuronalglial Tumors | |
Anaplastic ganglioglioma | III |
Tumors of the Pineal Region | |
Pineocytoma | I |
Pineoblastoma | IV |
Embryonal Tumors | |
Medulloblastoma | IV |
CNS primitive neuroectodermal tumors (PNETs) | IV |
Tumors of the Cranial and Paraspinal Nerves | |
Schwannoma | I |
Neurofibroma | I |
Meningeal Tumors | |
Meningeoma | I |
Anaplastic meningioma | II |
Hemangioblastoma | I |
Tumors of the Sellar Region | |
Craniopharyngioma | I |
Pituicytoma | I |
Histologic grading is a means of predicting the biologic behavior of a neoplasm. In the clinical setting, tumor grade is a key factor influencing the choice of therapies, particularly determining the use of adjuvant radiation and specific chemotherapy protocols. The WHO classification of tumors of the nervous system includes a grading scheme that ranges across a wide variety of neoplasms rather than a strict histologic grading system.10 Grade I applies to lesions with low proliferative potential and the possibility of cure following surgical resection alone. Neoplasms designated grade II are generally infiltrative in nature and, despite low-level proliferative activity, often recur. Some type II tumors tend to progress to higher grades of malignancy, for example, low-grade diffuse astrocytomas that transform to AA and glioblastoma. Similar transformation occurs in oligodendroglioma and oligoastrocytoma. The designation WHO grade III is generally reserved for lesions with histologic evidence of malignancy, including nuclear atypia and brisk mitotic activity. The designation WHO grade IV is assigned to cytologically malignant, mitotically active, necrosis-prone neoplasms. Examples of grade IV neoplasms include glioblastoma, medulloblastoma, and lymphoma.
Astrocytic gliomas, which are the most common primary brain tumors, arise from astrocytes, the supporting cells of the brain and spinal cord. The cytoplasmic processes that extend from the astrocytes contain a characteristic filamentous protein, glial fibrillary acidic protein (GFAP), which provides an immunohistochemical marker for these tumors. The WHO defines diffusely infiltrative astrocytic tumors with cytologic atypia alone as grade II (diffuse astrocytoma), those also showing anaplasia and mitotic activity as grade III (AA), and tumors additionally showing microvascular proliferation or necrosis as WHO grade IV (Fig. 21-3). Necrosis may be of any type; perinecrotic palisading need not be present.
Clinical Presentation
The presenting symptoms and signs of brain tumors are divided into those associated with a mass effect and increased intracranial pressure and those that are focal. The most common presenting symptom is headache, and approximately 20% of patients with supratentorial tumors present with seizures. Alterations in personality, mood, mental capacity, and concentration are frequently seen early in the clinical course.4 The focal neurologic symptoms and signs observed with supratentorial brain tumors are summarized in Table 21-2.
Anatomic Region | Symptoms or Signs or Both |
---|---|
Frontal lobe | Personality change |
Slowing of contralateral hand movements | |
Contralateral spastic hemiplegia | |
Mood elevation | |
Difficulty in adapting to new situations | |
Loss of initiative | |
Dysphagia + lip, tongue, and hand movements | |
Apraxia (if dominant lobe involved) | |
Bifrontal involvement | Bilateral hemiparesis |
Spastic bulbar palsy | |
Impairment of intellect | |
Lability of mood | |
Dementia | |
Primitive grasp, suck, and snout reflex | |
Temporal lobe | Impairment of recent memory |
Homonymous quadrantanopsia | |
Auditory hallucination | |
Aggressive behaviour | |
Non-dominant lobe | Minor perceptual problem |
Spatial disorientation | |
Dominant lobe | Dysnomia |
Impaired perception of verbal command | |
Fluent-Wernicke-like aphasia | |
Parietal lobe | Mild hemiparesis |
Mild to severe sensory loss | |
Homonymous hemianopsia | |
Visual inattention | |
Non-dominant lobe | Perceptual abnormalities |
Anosognosia | |
Apraxia for self-dressing | |
Dominant lobe | Alexia |
Dysgraphia | |
Other forms of apraxia | |
Occipital lobe | Contralateral homonymous hemianopsia |
Visual aberrations | |
Bilateral occipital involvment | Cortical blindness |
Thalamus or basal ganglia or both | Herniation syndrome |
Contralateral sensory abnormalities | |
Intermittent contralateral paresthesia | |
Neuropathic pain syndrome | |
Contralateral intention tremor | |
Semiballistic-like movement disorder | |
Hydrocephalus due to trapping of lateral horn of ventricle |
Routes of Spread
Malignant gliomas form as an expansile mass that conforms to the barriers of the cortical convolutions, deep nuclear structures, and adjacent myelinated nerve fibers.11 Microscopic examination typically shows a gradient of infiltrating tumor cells that decreases with the distance from the periphery of the mass. As they enlarge, malignant gliomas extend directly into adjacent lobes, infiltrate throughout the ipsilateral cerebral hemisphere, and disseminate along anatomically defined nerve fiber pathways. In some cases, individual cells, facilitated by the accompanying edema, may infiltrate for long distances from the main tumor mass. Multicentric gliomas are found in less than 5% of patients. Dissemination by seeding through the cerebrospinal fluid pathways occurs in approximately 10% of cases, but is usually a late event, often appearing at a time when its effects are inconsequential compared with those of the recurrent primary tumor mass. Metastases rarely arise outside the CNS.
Diagnostic Studies
Magnetic resonance imaging (MRI) is the modality of choice for diagnosis and evaluation of intracranial neoplasms. It is the ideal modality for initial preoperative diagnosis, including tumor extent, treatment planning, and image-guided therapies because of its multiplanar capability, anatomic detail, and superior resolution.12,13 The ability to characterize tissue allows for improved assessment of fatty, hemorrhagic, cystic, necrotic, and vascular components, as well as mass effect and location. In malignant gliomas, on T1-weighted sequence enhanced with gadolinium contrast, the tumor appears as an irregular, ringlike configuration that may surround a central area of necrosis (Fig. 21-4). On T2-weighted fluid-attenuation image recovery (FLAIR) images, the changes that reflect edema extend beyond the boundaries of contrast enhancement that may harbor microscopic extension. In fact, tumor may even be found in normal-appearing brain beyond the T2-weighted abnormality.
Imaging studies are performed within the first 48 hours after surgical resection to determine the presence of residual tumor and to provide a baseline for subsequent treatment. Enhancement resulting from surgical trauma may be indistinguishable from residual tumor even after a complete surgical resection. Postsurgical enhancement develops as early as the fifth postoperative day, peaks after 2 weeks, and may persist for months.14 Corticosteroids, which act to re-establish the blood-brain barrier, also have a profound effect on the area of enhancement, and diminution in the area of enhancement may be due to corticosteroids alone. To determine a radiographic response to therapy, patients should be on the same or a lower dose of corticosteroids than the dose at the time of the pretreatment scan.
Lacking with conventional MRI is an assessment of physiologic and functional information about the tumor. The gadolinium enhancement, as seen on T1-weighted MRI, reflects regions where there has been a breakdown of the blood-brain barrier. This may not be a reliable indicator of high-grade tumor because of the presence of nonenhancing tumor or contrast-enhancing necrosis.15 Similarly, T2-defined volume either overestimates or underestimates the microscopic or nonenhancing disease in a majority of patients. New MRI techniques, such as MR perfusion, MR diffusion, MR spectroscopy (MRS), and functional MRI (fMRI), can provide further physiologic characterization of the tumor related to hemodynamics, cellularity, and metabolism, respectively, and may be used to assess response to therapy.16
MRS is a noninvasive method to evaluate the malignancy of gliomas based on metabolite levels such as N-acetylaspartate (NAA), choline (Cho) compounds, total creatine (Cr), and lactate (Lac), or metabolite ratios such as Cho/NAA and Cho/Cr (Fig. 21-5). Spectroscopy also holds great promise in aiding target definition for radiotherapy treatment planning and evaluation of response to therapy and tumor recurrence.17 With the advantage of measuring tumor regional variations in abnormalities of metabolite levels, MRS has recently been used as an in vivo molecular imaging technique that assists in targeting and predicts response to radiation therapy for patients with brain gliomas.
In addition to MRS, brain fMRI has gained potential in clinical application recently as it can image the eloquent cortices, such as the motor cortex, the Broca area, the Wernicke area, and the visual cortex, which are difficult to identify on anatomic MRI. Incorporation of fMRI information into the radiotherapy treatment process can possibly allow a radiation oncologist to properly plan and deliver an adequate radiation dose while avoiding damage to the adjacent functional cortices in the treatment of gliomas.18
Dynamic MRI techniques can be used to measure important features of tumor vascularity in vivo, including the density and permeability of capillaries. Increased vessel numbers lead to higher regional cerebral (i.e., tumor) blood volume, which can be measured relative to healthy brain tissue by perfusion MRI techniques. Thus, relative cerebral blood volume provides analysis of capillary density in gliomas (Fig. 21-6). In adult astrocytomas, relative cerebral blood volumes correlate well with histopathologic grade and survival. A relative cerebral blood volume value of more than 1.75 is associated with the presence of high-grade tumor and with reduced survival in patients with gliomas.19
Prognostic Factors
A major contribution of the cooperative group brain tumor studies has been the identification of pretreatment characteristics that affect the outcome in patients with malignant gliomas. A nonparametric recursive partitioning technique was applied to an analysis of 1578 patients accrued to three successive Radiation Therapy Oncology Group (RTOG) trials.20 Age, histologic appearance, Karnofsky performance status (KPS), mental status, duration of symptoms, neurologic functional class, extent of surgery, and radiation dose were identified as significant partitioning covariates. As shown in Table 21-3, six patient classes were defined with median survival times ranging from 4.6 to 58.6 months and 2-year survival rates of 4% to 76%. These classes were used to define favorable (classes I and II, 12% of the patients evaluated), intermediate (classes III and IV, 43%), and poor (classes V and VI, 45%) prognosis subgroups. A subsequent reanalysis of the data in glioblastoma patients showed no statistical difference between class V and VI with a median survival time of 7.5 months.21 This information is important for interpreting correctly the results of studies comparing different treatment regimens and for assessing the potential of new therapeutic methodologies.
Table 21-3 Malignant Glioma Recursive Partitioning Analysis Classification
Class | Definition |
---|---|
I | Age <50, anaplastic astrocytoma, and normal mental status |
II | Age ≥50, KPS 70-100, anaplastic astrocytoma, and at least 3 months from time of first symptoms to initiation treatment |
III | —Age <50, anaplastic astrocytoma and abnormal mental status |
—Age <50, glioblastoma multiforme and KPS 90-100 | |
IV | —Age <50, glioblastoma multiforme, KPS <90 |
—Age ≥50, KPS 70-100, anaplastic astrocytoma and 3 months or less from time of first symptoms to start of treatment | |
—Age >50, glioblastoma multiforme, surgical resection, and good neurologic function | |
V | —Age ≥50, KPS 70-100, glioblastoma multiforme, either surgical resection and neurologic function that inhibits the ability to work or biopsy only followed by at least 54.4 Gy of RT |
—Age ≥50, KPS <70, normal mental status | |
VI | —Age ≥50, KPS <70, abnormal mental status |
—Age ≥50, KPS 70-100, glioblastoma multiforme, biopsy only, receiving less than 54.4 Gy of RT |
Standard Therapeutic Approaches
Surgery
The combination of surgery, radiation therapy, and chemotherapy represents the standard approach to the treatment of malignant gliomas. Generally, surgery is performed through an open craniotomy. The goals of surgery are to provide a histologic diagnosis, to alleviate intracranial hypertension and focal neurologic deficits resulting from a mass effect, and to permit rapid corticosteroid dose tapering.22 Furthermore, a large tumor mass left in the brain can serve as a nidus for cerebral edema after radiotherapy. The influence of surgical resection in malignant gliomas has been controversial. The aim of palliation of symptoms was always clear, but the survival advantage was debated. However the evidence suggests that patients with more complete resections designed to minimize the volume of residual tumor live longer and have an improved functional status compared with those who undergo a biopsy or partial resection only. An analysis of an RTOG database of 645 patients with malignant gliomas revealed a median survival of 11.3 months with total resection, 10.4 months with subtotal resection, and 6.6 months with biopsy alone.23 However, tumor size was not found to be a predictor of survival. Unfortunately, there are no well-conducted randomized clinical trials in high-grade gliomas that have tested the extent of surgical resection. A poorly done study on 30 elderly patients with radiologically obvious malignant glioma randomized to biopsy alone versus resection showed a borderline survival benefit with resection.24 Advances in neurosurgery, including diagnostic ultrasound, lasers, ultrasonic tissue aspirators, cortical mapping, functional imaging, and computer-assisted stereotactic laser techniques, have improved the ability of neurosurgeons to radically remove intracranial tumors.22
A closed-needle biopsy, using computed tomography (CT)- or MRI-coupled stereotactic techniques, may be indicated in many clinical settings. A biopsy is preferred for tumors located in functionally important or inaccessible areas of the brain. In addition, surgical resection is not practical for patients with significant tumor infiltration across the midline and around the ventricular system, or for those with diffuse, nonfocal lesions.22
Radiation Therapy
Randomized trials conducted by the Brain Tumor Cooperative Group (BTCG) and the Scandinavian Glioblastoma Study Group (SGSG) provided seminal evidence that external-beam irradiation favorably affects the outcome of malignant gliomas. BTCG trials 6901 and 7201 demonstrated a significant survival advantage for irradiated patients who received 50 to 60 Gy to the whole brain (in single daily fractions of 1.7-2.0 Gy, 5 days per week) either alone or with chemotherapy compared with those treated with either resection and supportive care only (P = 0.001) or with chemotherapy alone (P < 0.01).25,26 The median survival of patients receiving 60 Gy was 2.3 times longer than that observed for nonirradiated patients. Nearly 30% of irradiated patients in the SGSG trial maintained a full or partial working capacity, whereas the nonirradiated patients progressively deteriorated, and none regained their original performance level.27 Randomized trials of postoperative radiotherapy in gliomas is shown in Fig. 21-7. These studies were so convincing that virtually all patients with malignant gliomas receive adjuvant radiation therapy.
Chemotherapy
Until recently, the role of adjuvant chemotherapy in high-grade gliomas remained controversial. Historically, the nitrosoureas, especially carmustine (bis-chloroethyl-nitrosourea [BCNU]), was the most active single agent and no other drug or drug combination had been found to be more effective with the exception of the procarbazine, lomustine (CCNU), and vincristine (PCV) regimen.28 However, a retrospective analysis of RTOG data and a phase III trial conducted at the Medical Research Council (MRC) showed no benefit in survival with the PCV regimen. Similarly, no benefit of chemotherapeutic agents like tirapazamine, topotecan, paclitaxel (Taxol), interferon-β, and thalidomide were noted when used with standard radiation in RTOG phase II trials.28
Introduction of temozolomide has redefined the role of chemotherapy in gliomas and represents the new standard of care. It is a derivative of dacarbazine and an inactive prodrug that undergoes hydrolysis to active metabolite monomethyl triazeno imidazole carboximide when absorbed and results in methylation of guanine at the O6 and N7 positions at the deoxyribonucleic acid. It has several advantages over conventional chemotherapy agents. These include oral administration, rapid absorption, 100% bioavailability, ability to cross the blood-brain barrier, linear pharmacokinetics, and minimal delayed myelosuppression. A large, multicenter phase II trial done in recurrent gliomas refractory to PCV chemotherapy treated with temozolomide showed a 35% response rate (RR) and a 6-month progression-free survival (PFS) rate of 46%, and was subsequently Food and Drug Administration–approved for relapsed AA.29 Based on these responses, the European Organisation for Research and Treatment of Cancer (EORTC) conducted a phase III trial of 60 Gy of radiation with or without temozolomide at 75 mg/m2/day followed by six cycles of adjuvant therapy with temozolomide at 200 mg/m2 for 5 days per month in 543 patients.30 With a median follow-up of 28 months, the median overall survival improved from 12.1 to 14.6 months and the 2-year overall survival improved from 10.4% to 26.5% with the addition of temozolomide therapy.
Current focus of clinical development involves targeted therapies in glioma. Several agents have shown confirmed activity and are currently being investigated for integration in the initial management of malignant gliomas. These include antiangiogenic agents, tyrosine kinase blockers, inhibitors of Ras/MAPK pathways, and histone deacetylase inhibitors.31
Techniques of Radiotherapy
Most malignant gliomas are unifocal at the time of initial presentation, and after treatment the majority recur at or within 1 to 2 cm of their original location.32 Thus, limited treatment portals are used for malignant gliomas. Intracranial metastases that appear after partial brain irradiation do not affect the ultimate outcome, because they are nearly always accompanied by relapse at the primary tumor site. Whole-brain radiation therapy (WBRT) is commonly recommended for patients with multifocal tumors, but even for these lesions, relapses occur most frequently in the sites of known disease.32
The gross target volume (GTV) represents a three-dimensional (3-D) reconstruction of the location of the tumor, determined by merging data from contrast-enhanced CT scans and MRI studies. The integration of MRI into CT-based treatment planning provides complementary information for accurately defining the GTV. Little agreement exists as to the definition of the clinical target volume (CTV) or the planning target volume (PTV). A shrinking field approach is used in RTOG trials. The initial PTV (PTV1) encompasses the enhancing lesion (GTV) and edema (CTV) with a 2-cm margin. After 46 Gy of a conventionally fractionated treatment course, the PTV (PTV2) is reduced to include only the enhancing lesion with a 2.5 cm margin. Using the extent of edema to define the CTV has some limitations. The identification of peritumoral edema is subjective, and its volume may vary with corticosteroid dosage. An analysis of the patterns of failure using the RTOG-defined PTVs demonstrated that nearly all relapses occurred within the reduced PTV2 at the site of the enhancing tumor.33 Based on these data, we define the CTV by adding a margin of approximately 2.5 cm around the T1 contrast or a 1.5-cm margin around the FLAIR series. The PTV is determined by adding an additional 0.3- to 0.5-cm margin to the CTV to account for treatment uncertainties.
Intensity-modulated radiation therapy (IMRT) is an advanced form of 3D-CRT that uses inverse planning and computer-controlled radiation dose deposition. The advantage of precision delivery of radiation enables dose escalation and sparing of normal tissues. The brain IMRT treatment planning consists of immobilization using an Aquaplast face mask. A CT simulation with intravenous contrast is done with the acquisition of 1.5- to 3-mm thickness images. The previously obtained MRI data is superimposed on the CT images using the fusion program. GTV, CTV, and PTV are defined as in a 3D-CRT plan. All normal structures are outlined in three dimensions. User-defined constraints, including maximum and minimum dose constraints both for the PTV and the normal structures, are prescribed. As in 3D-CRT, noncoplanar beams are used. The plan consists of an intensity profile that is created for each beam. This is translated to leaf motion of the dynamic multileaf collimator using the inverse planning algorithm (Fig. 21-8). We have seen that the use of IMRT decreases the dose to the critical structures like optic nerves and brainstem when compared with the 3-D conformal plan.34 There is evidence that use of IMRT decreases the dose to the cochlea in children with medulloblastoma when used for the posterior fossa boost.
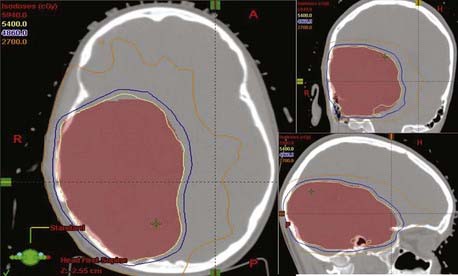
FIGURE 21-8 • Intensity-modulated radiation therapy treatment plan for a patient with high-grade glioma of right parietal region.
Special Issues With Radiation Management
Volume of Radiation
In the BTCG 8001 trial, patients were randomized to receive either 6000 cGy WBRT or 4300 cGy WBRT with 1720 cGy boost. No difference in survival or recurrence was noted.35 In a Japanese trial, patients were randomized to 4000 cGy WBRT with 1800 cGy boost or 5600 cGy local field radiation alone.36 Again, the 2-year survival rate was no different in this study (43% versus 39%), indicating that large-volume irradiation is unnecessary in high-grade gliomas.
Dose of Radiation
Radiation dose is another important consideration. In an MRC phase III trial, 444 patients were randomized to either 45 Gy in 20 fractions or 60 Gy in 30 fractions (1 : 2 randomization). A benefit of 60 Gy was seen in terms of 1-year survival (29% versus 39%) and the median survival (9 versus 11 months).37 In the RTOG phase III trial, patients received either 60 Gy WBRT or 60 Gy WBRT with a 10 Gy boost (Fig. 21-9). There was no benefit with the boost in terms of the median survival (9.3 versus 8.2 months).38 Researchers at the University of Michigan reported dose escalation to 80 Gy and 90 Gy with 3D-CRT and showed no difference in patterns of relapse or survival, indicating the absence of benefit with dose escalation beyond 60 Gy in malignant gliomas.39
Radiation Sensitizers
Multiple randomized trials conducted by the RTOG, the Brain Tumor Study Group (BTSG), and others have failed to show any survival benefit with misonidazole or other agents when compared with radiation therapy alone. Difluoromethylornithine, an inhibitor of polyamine synthesis, has not shown any benefit in a phase III clinical trial.40 Efaproxiral (RSR13), a synthetic allosteric modifier of hemoglobin, showed a median survival of 12.3 months when used along with 60-Gy radiation therapy in a phase II study, and is being presently tested in a phase III trial.41
Two halogenated pyrimidine analogues, bromodeoxyuridine (BUdR) and iododeoxyuridine, have been tested and in randomized trials have failed to show any benefit. Northern California Oncology Group data had shown a median survival of 252 weeks with BUdR with concomitant radiation and chemotherapy in AA compared with 82 weeks without BUdR.42 However, an RTOG trial of 60 Gy in 30 fractions combined with PCV chemotherapy with or without BUdR in 189 patients of AA had to be prematurely closed because of lack of benefit (1-year survival 68% versus 82%) and higher toxicity.43
Altered Fractionation
Again, multiple randomized trials conducted by RTOG, EORTC, and others have failed to show any survival benefit with hyperfractionation when compared with conventional radiation therapy alone.44 Similarly, several trials using different accelerated fractionation regimens have been reported; none have shown a survival benefit over conventional irradiation. An EORTC trial involving 60 Gy given in either conventional fractionation or with three fractions of 2 Gy given in a single day, 4 hours apart, in 340 patients showed no difference in survival or any increased toxicity.45 Doses of 64 and 70.4 Gy in 1.6-Gy, twice-daily fractions with BCNU tested in an RTOG trial showed no benefit.46 Shortening the treatment time has again failed to show any improvement.47 A Johns Hopkins trial of 30 Gy in 10 fractions followed by 2 weeks rest and then another 21 Gy in 7 fractions to reduced fields in 219 patients showed a survival similar to RTOG recursive partitioning analysis (RPA) groups I through VI patients.48
Brachytherapy
An earlier BTSG trial that randomized malignant glioma patients to 60 Gy radiation with or without iodine-125 (I-125) seed implant to 50 Gy showed a survival benefit with the addition of brachytherapy (median survival of 13 versus 16 months).49 Since the information was presented as an abstract only and never published, it remains difficult to establish that this approach clearly improves survival. However, a randomized trial done at Princess Margaret Hospital that randomized patients to 50-Gy external-beam radiation with or without temporary I-125 seed implant to 60 Gy did not show any survival benefit (median survival of 13.2 versus 13.8 months).50
Stereotactic Radiosurgery
Several retrospective trials have indicated that there is a possible survival advantage with the addition of a stereotactic radiosurgery (SRS) boost to high-grade gliomas. The RTOG conducted a randomized trial of conventional radiotherapy to 60 Gy and BCNU alone or preceded by a radiosurgery boost to 15 to 24 Gy in patients with GBM measuring 4 cm or less.51 However, the results were disappointing. The median survival (14 versus 13.7 months), 2-year survival (22% versus 18%), and 3-year survival (16% versus 8%), were similar with or without the boost. There was no improvement in any RPA class. No increased toxicities were seen with the addition of the SRS boost. Failures were still predominantly local (>90%).
Normal Tissue Reactions
Several adverse neurologic reactions may be observed in patients receiving cranial irradiation. They are classified into acute reactions, early-delayed reactions, and late-delayed injuries. With daily fractions of 1.8 to 2.0 Gy, the acute reaction most often presents as mild headache and nausea, beginning within a few hours after the first treatment and becoming progressively less severe with each succeeding fraction.52 The pathogenesis is thought to be increased cerebral edema caused by radiation-induced permeability changes in the blood-brain barrier. Corticosteroids may prevent or relieve most symptoms. Thus, if symptoms of increased intracranial pressure are present, patients undergoing cranial irradiation should be pretreated with corticosteroids (i.e., dexamethasone, 8-16 mg per 24 hours), administered for at least 48 to 72 hours before beginning treatment.
The early-delayed reaction (also called radiation encephalopathy) is characterized by transient, self-limited neurologic deterioration, somnolence, or focal encephalopathy.52 Early-delayed encephalopathy occurs in 15% to 40% of patients with primary or metastatic brain tumors. This reaction, which begins within 1 to 12 weeks after the completion of radiation therapy, usually peaks by 8 weeks after treatment and resolves spontaneously within the subsequent 4 months. Patients complain of headache, lethargy, and an exacerbation of their neurologic symptoms. At times, corticosteroid therapy and intensive medical support may be required. Declines in long-term memory within 1 to 2 months after irradiation followed by recovery 4 to 8 months later have also been observed.
Approximately 3% to 9% of patients irradiated for brain tumors develop clinically detectable focal radiation necrosis.53 Radiation necrosis may appear as early as 3 months after treatment, but usually develops within 1 to 2 years after treatment is completed. The breakdown of white matter may induce marked edema and mass effect. The symptoms of focal necrosis frequently recapitulate those of the tumor, leading the clinician to suspect recurrence. MRI may show a contrast-enhancing mass with extensive white matter alterations on T2-weighted images. Histopathologic findings are generally limited to the white matter and include focal coagulation necrosis and demyelination. Endothelial cell atypia and a unique form of fibrinoid necrosis of small arterial vessels are characteristic features that suggest the underlying pathophysiology.
Radiation necrosis is uncommon at doses less than 60 Gy with conventionally fractionated irradiation. The probability of necrosis increases with larger daily fraction sizes (2.2-2.5 Gy).37 For patients treated with hyperfractionated irradiation (twice-daily fractions of 1.2 Gy), the incidence of necrosis increased from 4.6% for 64.8 Gy to 19.2% for 81.6 Gy. Individual host factors, including the use of chemotherapy, may alter sensitivity and increase the risk of injury.
Corticosteroids may improve or stabilize the neurologic symptoms associated with the effects of radiation injury. Surgical resection is frequently beneficial to patients with favorably situated focal necrotic lesions who deteriorate neurologically and become steroid-dependent. There is no evidence that anticoagulation may lead to clinical improvement when surgery is not feasible. However, recent reports suggest a role for bevacizumab in the management of radiation-induced brain necrosis.54
Diffuse white matter injury develops in at least 40% of patients irradiated for intracranial neoplasms.52
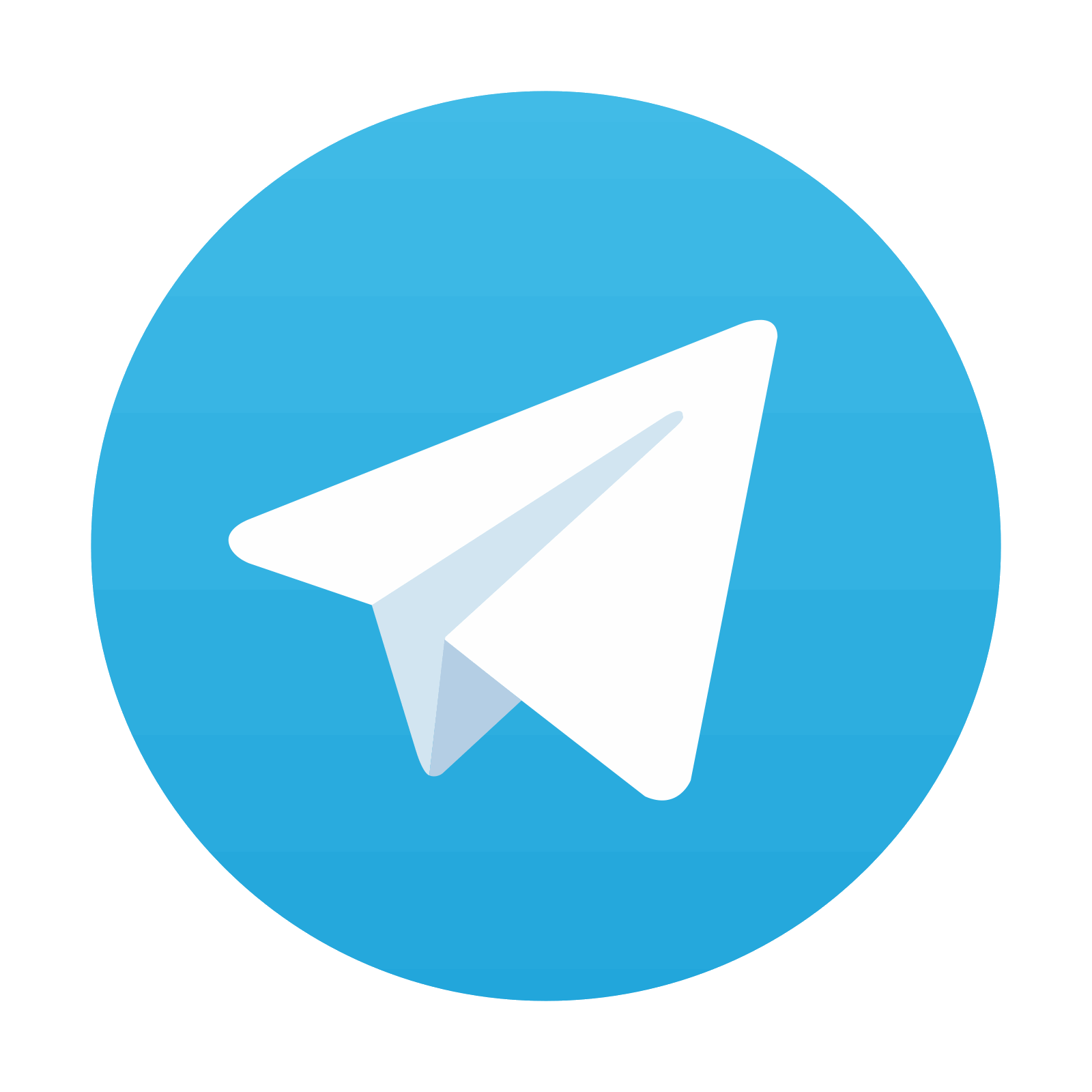
Stay updated, free articles. Join our Telegram channel
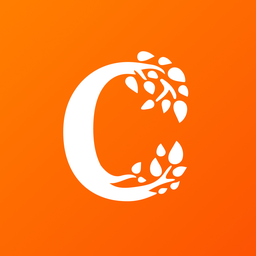
Full access? Get Clinical Tree
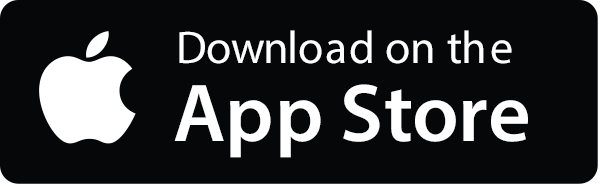
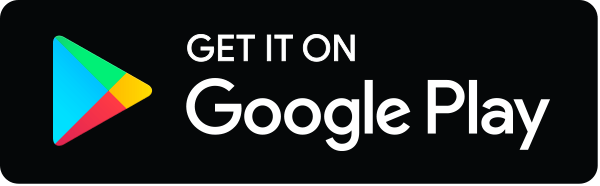