19 Kathleen M Botham The Royal Veterinary College The aim of nutrition research is to investigate the responses of the body to diet in order to determine optimal nutritional status in humans and animals – not only in the healthy adult, but at the various stages of development, including growth, reproduction and ageing – and also to study the relationship between diet and disease. It was first recognised in the late eighteenth century that, in addition to the deleterious effects of insufficient food in the diet (i.e. under-nutrition or starvation), components of the diet (which we now call nutrients) are necessary for the proper functioning of the physiological processes of the body and for the prevention of disease. Since then, nutritionists have used intervention studies, in which human healthy subjects, patients or animals (see Chapter 3) are fed a particular food or dietary component and the effects on their physiology and metabolism are assessed, and epidemiological (or population) studies, which observe the patterns of dietary intake and their relationship to health and disease in defined populations (see Chapter 2). These approaches have been very successful and have led to great strides in the understanding of how nutrients influence health. However, if we are to comprehend fully the mechanisms by which nutrients affect physiological responses, it is essential to study the events occurring at the cellular and molecular level that lead to changes in the functions of cells and tissues. Since the amount of information of this kind that can be obtained from epidemiological or intervention studies is limited, cellular models are widely used for these investigations. Together with the advances in scientific disciplines such as genetics and molecular biology that have occurred in the last few decades, such models have made it possible for scientists to dissect out the intracellular pathways by which cells alter their function in response to signals from their environment. The use of cellular models, therefore, is crucial for the further advancement of nutritional science. For the purposes of this chapter, cellular models will be defined as those involving the use of primary cells (i.e. cells derived directly from humans or animals), immortalised cell lines, tissue microstructures (e.g. pancreatic islets), tissue explants and isolated perfused organs. These experimental systems are currently being used or have the potential to be used in many different aspects of nutrition research, including: Experimental systems may be described generally as in vivo, a Latin term meaning literally ‘within the living’, ex vivo (‘outside the living’) or in vitro (‘in glass’); see Table 19.1. Table 19.1 In vivo, ex vivo and in vitro experimental systems. The advantage of using ex vivo or in vitro as compared to in vivo systems is that the immense complexity of the whole animal, which makes the study of particular cell and organ functions or responses extremely difficult, is simplified so that particular effects can be investigated more easily. Ex vivo experiments have the additional advantage of retaining the tissue architecture, but the disadvantage that they are inherently short term, while in vitro experiments allow scientists to drill down to the molecular mechanisms that enable cells to function. The main disadvantage of both ex vivo and in vitro approaches is, of course, that the studies are done outside the natural environment of the tissues or cells, and thus great care needs to be taken in applying the results to the in vivo situation. In nutrition research, for example, nutrients producing a particular desirable response in cells in culture in vitro may fail to do so in vivo because they do not arrive at the target tissue in sufficient quantities or because they have adverse effects on other cell types. Cellular models may be employed in ex vivo and in vitro systems, but by definition they are not used in studies in vivo. Cultured cell models can be divided into two general types, primary cells and immortalised cell lines (Table 19.2). Table 19.2 Types of cellular models. Primary cells are isolated directly from humans (mainly blood cells, but other cell types can also be obtained from biopsy samples) or experimental animals by gentle disruption of the target tissue in various ways that avoid destruction of cellular integrity (see later in this chapter). Some types of primary cells grow well in culture (e.g. fibroblasts), while others can be maintained for some days, but do not divide (e.g. hepatocytes). Primary cells have the advantage that they usually retain the physiological features they demonstrate in vivo, the results obtained are generally relatively easy to interpret and they are cost effective, as much smaller amounts of reagents are required than for in vivo experiments. However, some specialised cell types tend to become dedifferentiated (i.e. lose their specialised functions) in culture: for example chondrocytes (the cells that produce cartilage proteins) do not retain the chondrocyte phenotype, but revert to fibroblast-like cells, and the expression of liver-specific genes is progressively lost in cultured hepatocytes. Strategies have been designed to limit dedifferentiation, but they have met with limited success. A more generally applicable disadvantage is that, with the exception of stem cells, all primary cells are only able to survive in culture for a limited time. After a certain number of cell divisions the cells become senescent and stop dividing. This phenomenon is termed the Hayflick limit, after the scientist who first observed that human fetal cells divide only 40–60 times before entering the senescence phase. The shortening of telomeres in the DNA of the cells is believed to be the reason why further cell division eventually becomes impossible. Stem cells have the ability to differentiate into different types of specialised cells and are found in embryonic tissue and in adults in bone marrow, adipose tissue and blood. They can divide indefinitely in culture as well as in vivo. Unlike primary cells, immortalised cell lines are able to undergo unlimited cell division without becoming senescent. Immortalisation happens in vivo when cells mutate, become cancerous and proliferate in an uncontrolled way. Cancer cells cultured in vitro continue to divide indefinitely, and this makes them a useful tool for nutrition research. An example still widely used today is the HeLa cell line, which was originally derived from a human cervical cancer in 1951. More examples of immortalised cell lines are shown in Table 19.3. Table 19.3 Some immortalised cell lines. *Can be differentiated into macrophages by treatment with phorbol ester in vitro In addition to obtaining cell lines from naturally occurring cancers, it is also possible to generate them in the laboratory by genetic manipulation (Figure 19.1). This can be done in a random way by treating cultured cells with a known mutagen, then selecting continuously dividing cells. More targeted approaches, however, aim to introduce into the cells genes for specific proteins that influence cell division using a recombinant virus vector, such as adenovirus or a retrovirus. Proteins that have been used effectively to transform primary cells into immortalised cell lines include telomerase, an enzyme that lengthens telomeres and thus enables cell division to continue indefinitely, and viral genes, which interfere with the normal cell cycle. For example, telomerase transfection has been used to immortalise primary human skin fibroblasts, retinal cells and endometrial stromal cells, and adenovirus technology was used to generate the human embryonic kidney (HEK) 293 cell line by the introduction of a viral gene for a protein that alters the cell cycle so that cell division is promoted. Antibody-producing B lymphocytes can also be transformed into cell lines by fusion with a cancerous B cell (myeloma) forming a hybridoma, a technique that is used for the production of monoclonal antibodies. Figure 19.1 Generation of immortalised cell lines in the laboratory. Cells may be transfected with genes such as the Simian virus large T and small t tumour antigens (SV40T), which influence the cell cycle or the catalytic subunit of human telomerase reverse transcriptase (hTERT). The genes are incorporated into the genome and stably expressed, allowing unlimited cell division. The use of immortalised cell lines in nutrition research has the advantage that, because they continue to divide in culture indefinitely, they provide an unlimited quantity of homogenous, genetically identical cells, and so it is possible to carry out large numbers of experiments easily and relatively cheaply. The disadvantage of this type of model, however, is that because mutation is required for immortality, the cells may show significant differences from the primary cells from which they were derived, and highly specialised functions may be lost. It is important, therefore, to be aware of these limitations when interpreting the results. An explant is a small block of tissue that is taken from an animal or human biopsy sample under sterile conditions and maintained in an artificial medium ex vivo or in vitro (Table 19.2). Explant culture has the advantage that the cell architecture and extracellular matrix remain intact in the tissue blocks, thus enabling their function to be studied in an environment that more closely resembles in vivo physiology than that in cultured isolated primary cells. Examples include the use of animal cartilage explants to study osteoarthritis and ileal tissue explants to study the function of intestinal cells. However, because of the difficulty of maintaining the viability of cells in the centre of the block, explants are of necessity very small (no more than a few millimetres long), so the amount of material that can be obtained is limited, and this technique is much less widely used than cell culture models in human and animal studies. In addition to their use to study tissue function, cultured explants are also employed in the isolation of primary cells, which can then be grown on in the absence of the tissue block (see later in this chapter). Adipose tissue explants are commonly used in this way to provide adipocytes for the study of the causes of obesity. Perfused organ systems use animal organs such as the liver and heart, which are isolated from the body and perfused through their physiological vascular system using an oxygenated perfusate, or even sometimes whole blood (Table 19.2). This has the advantage that a particular organ in its entirety, with its various cell types embedded in the natural tissue architecture, can be studied in the absence of the influence of other organs or factors such as hormones that circulate in the whole animal. The disadvantages are that perfusion systems are necessarily rather complex and the organ can only be kept alive for a limited time, usually some hours. As well as the liver and heart, organs that are widely used in perfusion studies include the kidneys, lungs, intestine and blood vessels such as the aorta. Rodent such as rats, mice and guinea pigs are often employed as donors, but organs from larger species such as pigs and sheep have also been used successfully. Cellular models may be employed in ex vivo and in vitro systems, but by definition they are not used in studies in vivo. We can divide the experimental approaches involving cellular models that are likely to be used in nutrition research into two main types. The first type resembles an intervention study, in that human volunteers (either healthy or after diagnosis of disease) or experimental animals (normal or disease models caused by mutation – e.g. the ob/ob obese mouse, a model for obesity and type II diabetes – or induced by chemicals/drugs – e.g. small doses of carbon tetrachloride used to induce liver disease – or diet – e.g. the use of a high-carbohydrate, high-fat diet to induce obesity) are given the test food or nutrient in the diet (or for acute studies in a test meal), and tissues are then taken for cell culture or organ perfusion (Figure 19.2). For obvious reasons, in experiments involving humans this approach is mostly limited to blood cells, but sometimes cells can be isolated from biopsy samples (e.g. hepatocytes can be isolated from liver biopsy samples). Basic cell functions and their responses to external factors added to the cell medium or organ perfusate can then be investigated and the effects of the test nutrient on the cells/tissues determined by comparing the results with those observed in cells/tissues from subjects/animals given a control diet or test meal. In this way, information may be gained about how nutrients taken in the diet influence normal cell function and thus may have health benefits, and also about how they may protect against or retard disease processes. Figure 19.2 Cellular models in nutritional intervention studies. The second, simpler approach is entirely in vitro and may involve isolated perfused organs, primary cells or immortalised cell lines (Figure 19.3). In this case the effects of a nutrient on cell/tissue function are assessed directly by adding it to the perfusate or cell culture medium of primary cells harvested from humans or experimental animals (either healthy or disease models, as earlier) or to cell lines. This type of study has the advantage of in vitro systems in being easy to use and providing large quantities of data relatively inexpensively, but the disadvantage that the normal physiological processes of digestion and absorption are bypassed, and thus any changes observed may not reflect what happens in vivo. Figure 19.3 In vitro experimental systems in nutrition research. For culture in vitro, most primary cells must first be released from the extracellular matrix with no, or minimal, damage to the plasma membrane, as otherwise they will not be able to survive. Leucocytes (white blood cells) are an important exception to this, as they can be isolated directly from the blood. Enzymes such as collagenase, which attack the matrix protein collagen, or other general proteases such as trypsin or pronase are often used for the release of cells from tissues. The tissue is minced or gently homogenised before treatment with the enzymes. Perfusion of the organ with collagenase can also be used with highly vascularised tissues, and this approach has been very successful for the the isolation of hepatocytes from the liver. Since calcium ions play an important role in cell–cell adhesion in the tissues, a calcium chelator (an agent that binds to calcium ions) such as ethylenediaminetetraacetic acid (EDTA) is often used in conjunction with the proteases. An alternative method avoiding the use of enzymes that may damage the cell membrane is the use of tissue explant culture (see earlier). In this technique, single cells that grow out from a small piece of tissue maintained ex vivo are harvested, then grown and used in a culture system. Once the cells have been released from the extracellular matrix or isolated from the blood, another step is often required to separate the desired specialised cell type from the mixed population usually present in the cell suspension. This is important, because just a few fast-growing cells such as fibroblasts can rapidly overgrow other cell types in culture. Cells may be separated on the basis of their: Figure 19.4 Principle of magnetic activated cell sorting (MACS) and fluorescence activated cell sorting (FACS). (a) MACS. Cells are treated with magnetic beads carrying antibodies, which bind to antigens on the cell surface. A strong magnetic field is applied and cells expressing the antigen stay attached to the particles, while non-expressing cells remain unbound and can be removed. In positive selection cell types that remain bound to the particles are harvested, while in negative selection the unwanted cells bind, leaving the required cell type free in the solution. (b) FACS. Cells are treated with a fluorescent antibody, passed singly through a laser beam, then given a positive or negative charge and separated by the application of an electric field. The detectors allow analytical data to be collected on the size and granularity (1) or fluorescence intensity (2) in the cells. Application of these techniques results in near homogenous preparation of specific primary cell types, which can then be grown or maintained in culture. Immortalised cell lines obtained either from naturally occurring cancers or by laboratory manipulation (see earlier) retain their viability when stored at –80 °C after freezing in liquid nitrogen, and thus can be recovered and grown up when required. The techniques used for cell culture are similar for both primary cells and immortalised cell lines. The cells are placed in flasks or dishes and kept at the appropriate temperature in an incubator in which the atmosphere can be controlled. Typical conditions for mammalian cells are a temperature of 37 °C and an atmosphere of 5% carbon dioxide/95% air. The culture medium generally consists of a mixture of salts, amino acids, vitamins, growth factors, antibiotics and antifungal agents at a defined pH (usually 7.4) with glucose as a fuel source, but the exact composition varies depending on the cell type under study. Table 19.4 shows the contents of a typical culture medium suitable for most types of cells. Media are also commonly supplemented with a small amount of animal serum (e.g. fetal calf serum) to provide a physiological profile of growth factors. In addition, a pH indicator such as phenol red is often included so that any pH changes can be detected. Table 19.4 Contents of Dulbecco’s modified Eagle’s medium, one of the most common medium formulations, used for the culture of all types of cells. Antibiotics such as penicillin and streptomycin are also often added to inhibit bacterial growth. Clearly, it is important to be sure that the cells used in culture are viable at the start and throughout the experiment. Typically, cell preparations with less than 90% viability are discarded. Viability may be assessed in a number of different ways. One of the most common is the Trypan blue exclusion test. Since the blue dye does not cross the membrane of intact cells, dead cells appear blue, while live cells remain colourless. Other membrane integrity tests include the use of propidium iodide, which can distinguish between normal, necrotic and apoptotic cells, and the assay of lactate dehydrogenase, an enzyme that leaks into the medium when the cell membrane is damaged. The spectrophotometric MTT assay, which measures the activity of enzymes that reduce the tetrazolium dye MTT to the purple-coloured formazan, is also widely used. The two main types of culture system are suspension, where cells float freely in the culture medium, and adherent, where cells attach to a suitable surface, usually in a single layer. As indicated earlier, hematopoietic (blood) cells grow in suspension, but most animal tissue-derived cells are adherent, meaning that they attach, spread and grow when provided with a suitable hydrophilic, negatively charged surface. Plastic dishes that have been chemically treated to generate this kind of surface are, therefore, essential for most cell culture systems. They are termed tissue culture treated and are widely available commercially.
Cellular Models in Nutrition Research
19.1 Introduction: Why use cellular models in nutrition research?
19.2 Cellular models in ex vivo and in vitro experimental systems
System
Literal meaning
Type of experiment
Advantages
Disadvantages
In vivo
Within the living
Whole animal
Physiological conditions maintained throughout
Complexity makes interpretation of results difficult
Ex vivo
Outside the living
Perfused organs, tissue segments and explants maintained in the laboratory outside the organism
Enables specific tissue functions to be studied while retaining tissue architecture and extracellular matrix
Only short-term experiments possible
Outside natural environment
In vitro
In glass
Isolated cells or cell components maintained in culture dishes or (subcellular organelles or proteins) used in test tubes
Enables the study of cell function at the molecular level
Interactions with other cells/tissues excluded
Outside natural environment
19.3 Types of cellular models
Cultured cells
Model type
Origin
Advantages
Disadvantages
Primary cells
Isolated directly from animal tissue or human blood/biopsy samples
Retain in vivo physiology
Relatively inexpensive
Limited life in culture (Hayflick limit)
Extracellular matrix and tissue architecture lost
May not divide in culture
May lose tissue-specific function in culture
Immortalised cell lines
Cells derived from cancerous tumours or laboratory manipulation
Unlimited divisions in culture
Unlimited quantity of genetically identical material available
Relatively inexpensive
Often show significant differences from parent cell type
Specialised tissue function may be lost
No extracellular matrix or tissue architecture
Tissue explants
Small blocks of tissue from animals or human biopsy samples
Maintain tissue architecture and extracellular matrix
Can be used to obtain cultures of primary cells
Limited amount of material available
Often only short-term experiments possible
Isolated perfused organs
Directly from animals by cannulation of suitable blood vessels
Maintain whole organ with various cell types in natural tissue architecture
Complex experimental systems required
Only relatively short-term experiments possible
Primary cells
Immortalised cell lines
Name
Species
Origin
Cell type
HeLa
Human
Cervical carcinoma
Epithelial
THP-1
Human
Acute myeloid leukaemia
Monocytes/macrophages*
HEK293
Human
Transformation of embryonic kidney cells using adenovirus
Kidney epithelial
CaCo-2
Human
Colorectal adenocarcinoma
Intestinal epithelial cells (enterocytes)
HepG2
Human
Hepatocellular carcinoma
Hepatocytes
MCF-7
Human
Breast adenocarcinoma
Mammary epithelial
CHO
Hamster
Chinese hamster ovary
Epithelial
RAW264.7
Mouse
Abelson murine leukaemia virus-induced tumour
Macrophages
McA-RH7777
Rat
Hepatoma
Hepatocytes
CV-1
African green monkey
Adult kidney cells transformed with Rous sarcoma virus
Fibroblasts
COS-7
African green monkey
CV-1 cells transformed with simian virus 40
Fibroblast-like
Tissue explants
Isolated perfused organs
19.4 General approaches in nutrition research
19.5 Cell culture methods
Cell isolation
Cell culture
Salts
Amino acids
Vitamins
Others
Calcium chloride
Ferric nitrate
Magnesium sulphate
Potassium chloride
Sodium bicarbonate
Sodium chloride
Sodium monohydrogen phosphate
Arginine
Cystine
Glycine
Histidine
Isoleucine
Leucine
Lysine
Methionine
Phenylalanine
Serine
Threonine
Tryptophan
Tyrosine
Valine
Glutamine
Choline
Folic acid
Myo-Inositol
Niacinamide
Pantothenic acid
Pyridoxal
Pyridoxine
Riboflavin
Thiamine
Glucose
Pyruvic acid
Phenol red (if a pH indicator is required)
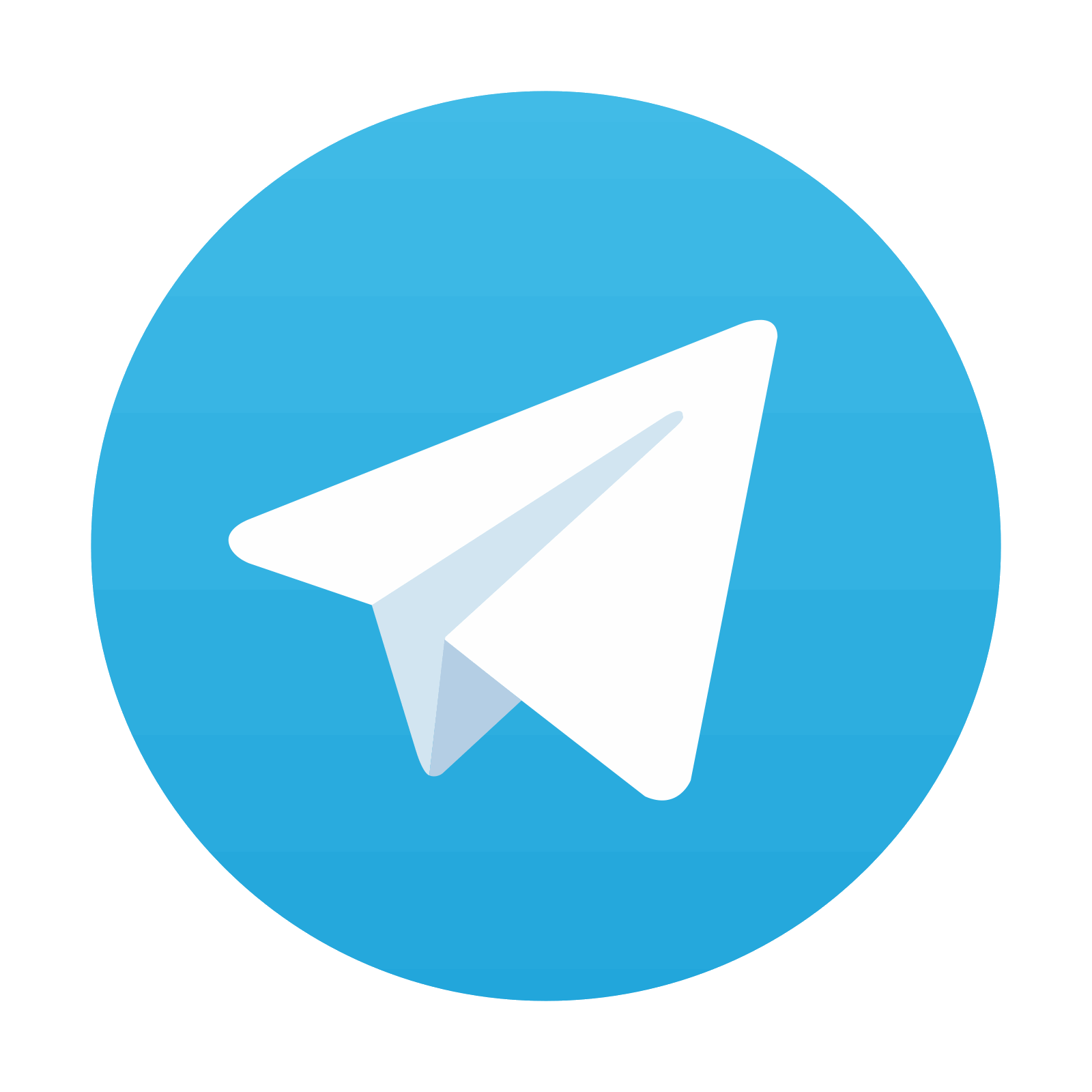
Stay updated, free articles. Join our Telegram channel
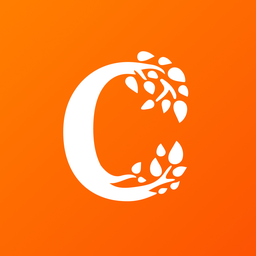
Full access? Get Clinical Tree
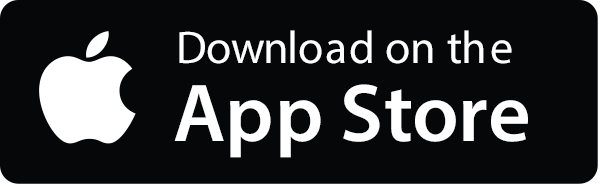
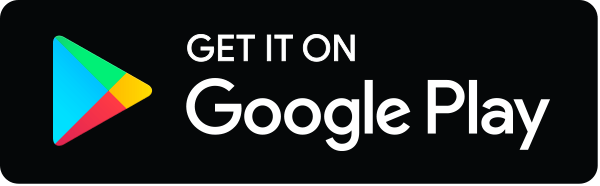