Fig. 13.1
Estimated market for autologous cell therapy (http://www.marketsandmarkets.com/PressReleases/stem-cells-market.asp)
Despite a humongous need for cell therapy for diabetes, only a small portion has been addressed, in terms of a consistent replenishment of damaged β cells. However, all the research work that have been carried out, till date, indicate relatively long-term graft survival rates, when compared to whole pancreas transplantation (Robertson et al. 2000). Long survival of the islet transplant graft is attributed to the better engraftment and immune response, as compared to whole pancreas transplantation.
Goals for obtaining an inexhaustible supply of functional β islet cells are achieved with varying degrees of success. Differentiation of human pluripotent (embryonic and induced pluripotent) cells, of respectively embryonic and adult origin, toward β cell lineage and direct reprogramming of the non-endocrine pancreatic cells, into functional insulin-producing cells, are the steps taken. Other cell types like conditioned lymphocytes, mononuclear cells, or a combination of cell types including hematopoietic, pluripotent, and multipotent ones are reportedly safe and feasible cell therapy options for diabetes (Jarajapu and Grant 2010).
Mesenchymal stem cells (MSCs), due to their property to secrete trophic factors, upon combination with other cell types, differentiated into functional β cells, and have thus provided added efficacy to cell therapy for diabetes (Jafarian et al. 2014).
13.2 Classical and Novel Approaches: Surgical and Non-surgical Approaches to Treat Diabetes and Respective Underlying Pathophysiology
With the worldwide epidemic of obesity, there has been epidemic of type II diabetes, which affects more than 370 million people globally (International Diabetes Federation Update 2012). Hence, treating insulin resistance is a major approach to control the epidemic of type II diabetes.
In order to maintain homeostasis, under insulin resistance conditions, β-cells initially increase the insulin output, thereby maintaining normal glucose tolerance. However, with further increase in insulin resistance, as in the case of obesity, the increased insulin output by the β-cells becomes insufficient to maintain normoglycemic conditions. Insufficient insulin production, under insulin resistance conditions, is often referred as β-cell dysfunction. β-cell dysfunction has a genetic as well as environmental component. The environmental component, in terms of diet and obesity, is playing havoc in causing type II diabetes. Research results have established an important correlation between hexoses and other carbohydrates, amino and fatty acids, insulin resistance, and β-cell dysfunction in modern times (LeRoith 2002).
Dietary imbalances in carbohydrates and fatty and amino acids have been proved to govern insulin resistance and β-cell dysfunction. Increased intakes of saturated dietary fat contribute to the development of obesity, insulin resistance, β-cell dysfunction, and glucose intolerance (Hu et al. 2001). Accordingly, modern strategies are being developed to maintain an optimum balance between the factors that govern β-cell dysfunction. Slowing down the β-cell dysfunction is one of the important strategies (Weir and Bonner-Weir 2004). Another one is to control type II diabetes via obesity reduction by bariatric surgery (Dixon et al. 2011). Finally, cell therapy is also resorted to cure in morbidly obese individuals suffering from type II diabetes.
Oral and injectable drugs aim to maintain the plasma concentrations of glucose at normoglycemic levels, thereby preventing diabetes-related complications. Examples of such drugs are sulfonylurea, biguanide, α-glucosidase inhibitors, and peroxisome proliferator-activated receptor γ (PPAR γ) agonists. Drugs like α-glucosidase inhibitors pose their effect by slowing the glucose absorption by the gastrointestinal tract (GI), thereby delaying the degradation of complex carbohydrates in the GI tract (van de Laar et al. 2005). One example of α-glucosidase inhibitors is pramlintide (Younk et al. 2011).
Various oral drugs available in contemporary times, and approved for the treatment of type II diabetes, are second-generation sulphonylureas, for example, glibenclamide (also known as glyburide) and glimepiride. Biguanide antidiabetics include metformin. Pioglitazone and rosiglitazone are PPAR γ agonists. α-glucosidase inhibitors are represented by acarbose, miglitol, and voglibose. One should also mention dipeptidyl peptidase (DPP4) inhibitors like algopiptin and linagliptin, sodium–glucose co-transporter 2 inhibitors like canagliflozin and dapagliflozin, and bile acid binding resins like colesevelam. Another oral drug named bromocriptine has been used as an antidiabetic, since it is believed to restore glucose metabolism via central nervous system by regulating the circadian rhythm (Weyer et al. 1999).
Earlier, insulin was the only injectable drug available for the treatment of diabetes. In current times, other drugs have been approved. They encompass islet amylin polypeptide analogues like pramlintide and glucagon-like peptide 1 (GLP1) receptor agonists like exenatide, liraglutide, and lixisenatide. Besides, there have been advancements in types of insulin used as injectables: rapid or short acting insulin, for example, soluble or regular insulin, insulin aspart, insulin glulisine, insulin lispro, and insulin zinc amorphous; intermediate acting insulin, for example, isophane insulin/NPH insulin and insulin Zinc/Insulin lente; and long-lasting insulin, such as insulin zinc crystalline/insulin ultralente, insulin detemir, and insulin glargine (Kahn et al. 2014).
Therapies aiming at improvement of β-cell mass, or increasing β-cell secretory function, are either at research stage or in clinical trials. GPR40/free fatty acid receptor 1 (FFAR1) agonists are known to increase β-cell secretory function. TAK-875 is such an FFAR1/GPR40 agonist, which is in phase III clinical trial (Burant et al. 2012). Similarly, agonists of the receptor GPR119, located on β-cells and intestine, result in improved β-cell secretory function via release of incretin (Tolhurst et al. 2012). Betatrophin, a probable revolutionary compound, is a liver-derived protein first identified by Douglas Melton’s group last year, resulting in increase of β-cell mass (Yi et al. 2013). Other approaches are decreasing the effect of glucagon, using glucagon receptor antagonists or glucagon antibodies (Gelling et al. 2003), reducing the hepatic production of glucose, by increasing the activation of glucokinase (Meininger et al. 2011), and increasing insulin action by inhibition of PTBIB (Ma et al. 2011).
Bariatric surgeries (laparoscopic adjustable gastric banding, laparoscopic Roux-en-Y gastric bypass, and laparoscopic sleeve gastrectomy) are surgical interventions aiming at obesity reduction, yet these interventions have successfully attenuated type II diabetes (O’Brien et al. 2013; Courcoulas et al. 2014; Svane and Madsbad 2014). They have reportedly increased insulin sensitivity and gut hormone response, insulin secretion via improved β-cell mass, by tenfold increase in glucagon-like peptide 1 (GLP-1), and also reduced the mortality related to type II diabetes (Svane and Madsbad 2014). Also, they resulted in improved metabolism of patients (Raghow 2013). The downside are complications related to long-term nutritional deficiencies.
Cell-based therapies have different approaches, thus being fit for the treatment of type I diabetes, which results in autoimmune destruction of β-cells and requires intense attention. In contrast, type II diabetes can be managed by drugs and lifestyle changes. However, extreme cases of type II diabetes resulting from morbid obesity would also need cell therapy for diabetes.
Pancreas transplantation, islet transplantation, and β-cell transplantation involve surgical interventions (Chhabra and Brayman 2014), while intravenous injections of bone marrow mesenchymal stem cells, to mitigate diabetes, involve non-surgical procedures (Hao et al. 2013). Isolated islet transplantation is already less invasive than whole pancreas transplantation and hence could represent a safe, effective, and definitive treatment option for diabetes (Berney and Johnson 2010).
Isolated islet transplantations involve considerable improvements in the levels of glycated hemoglobin (HbA1c), reduced incidence of debilitating hypoglycemic episodes in transplanted individuals, and improved insulin sensitivity, glucose disposal, and free fatty acid clearance (Barton et al. 2012). Furthermore, preprocedural complications of islet transplantation have 20-fold lower risk of morbidity, reportedly as compared to whole pancreas transplantation (Barton et al. 2012). In a preclinical model of diabetic rats, tail vein infusions of MSC, a less invasive method of administration, have been successfully used for the treatment of diabetes (Si et al. 2012). Taken together, cell therapy might prove to be the best approach to treat type I, and extreme cases of type II diabetes, as it is less invasive and more efficacious.
13.3 Cell Therapy for Diabetes: A Superior Holistic Approach for Continued Research and Treatment
In type I diabetes, β cells are mostly destroyed, and in type II diabetes β cell numbers may be reduced by 40–60 %. The proof-of-concept studies say that cellular transplants of pancreatic islets, which contain insulin-secreting β cells, can reverse the hyperglycemia of type I diabetes. There is now a need to find an adequate source of islet cells. Human and mouse pluripotent stem cells (human embryonic and human induced pluripotent) have been successfully differentiated into functional insulin-producing β-cells (Jiang et al. 2007a, b; Kroon et al. 2008; Bose et al. 2012; Jeon et al. 2012). From a research viewpoint, induced pluripotent stem cells (iPSCs) can also be generated from patients with diabetes to allow studies of the genomics and pathogenesis of the disease (Maehr et al. 2009).
Some alternative approaches for replacing β cells include finding ways for enhancing the replication of existing β cells, stimulating neogenesis (the formation of new islets in postnatal life), and reprogramming of pancreatic exocrine cells to insulin-producing cells. Stem-cell-based approaches could also be used for modulating the immune system in diabetes or to address the problems of obesity and insulin resistance in type II diabetes.
In accordance with the strategy of immune modulation, cord blood-derived multipotent stem cells have been used, to target insulin resistance in type II diabetic patients, and are already in phase I/II/clinical trials (Zhao et al. 2013). A list of all possible sources to obtain β-cells for cell therapy for diabetes include (1) insulin-secreting cell lines, (2) engineered non-β-cells for gene therapy, (3) β-cells or islets from other species as xenografts, (4) tissue stem cells from the pancreas, (5) bone marrow, (6) liver, (7) neural origin, and (8) pluripotent stem cells (PSCs), both embryonic and induced pluripotent (Jones et al. 2008).
In the case of type I diabetes, cell therapy is undoubtedly the ultimate solution. However, in case of type II diabetes, cell therapy can also be utilized to address the associated factors like metabolic improvement and restoration of insulin sensitivity. Indeed, cell therapy for type II diabetes does not mean pancreatic β-cell transplantation, under all situations. It can be conducted in the form of immune modulation via other kinds of stem cells, like bone marrow or cord blood MSCs. An elegant example to reverse type II diabetes is by using cord blood MSC to ameliorate insulin resistance (Zhao et al. 2013).
Use of immune/metabolism modulating stem cells can also give clues toward cases of insulin resistance that never progress toward a diabetic state. Immune modulation of type I diabetes can also be beneficial for β-islet regeneration (Abdi et al. 2008). Furthermore, as reported by Abdi et al., mesenchymal stromal cells have the ability to promote immunity and could prevent autoimmune destruction of β-cells in type I diabetes.
Cell therapy could also be applied to treat diabetic complications, such as atherosclerosis and microvascular disease. Treating diabetes and handling associated comorbidities are equally important. In this scenario, cell therapy from iPS cells, mesenchymal, cord blood, and adipose-derived stem/non-stem cells are prospective candidates.
13.4 Various Kinds of Available or Feasible Cell Therapies for Diabetes in Clinics and Research
13.4.1 Human Cadaveric Whole Pancreas or Islet Transplantations as Cell Therapy for Diabetes
Transplantation of whole pancreas was the very first kind of cell therapy administered for type I diabetic patients. Whole pancreas poses comparatively fewer complications related to surgery and ensures a permanent solution for hyperglycemia. Islet transplantation, a less invasive procedure, encompasses transplantation of islets in the portal vein of the recipient’s liver (White and Manas 2008; Merani and Shapiro 2006; Ricordi and Strom 2004). Islet isolation involves mechanical as well as enzymatic separation of islets from other tissues of the cadaveric pancreas. Islets have a different density than the acinar tissue, ensuring enrichment of an islet population following centrifugation. Indeed, automated methods for obtaining pure population of human islets have been in vogue since more than two decades (Ricordi et al. 1988).
Islets purified by density gradient centrifugation can be administered intraportally into the liver of the patient. Such islets have the capacity to home and revascularize in few weeks’ time, thereby replenishing the islet cell pool of diabetic patients (Korsgren et al. 2008). Islet transplantation incorporates a true amalgamation of isolation method (Ricordi et al. 1988), and steroid free immunosuppression protocol, for long-term graft survival (Shapiro et al. 2000). However, initially the long-term graft survival was poorer than expected, and only 20 % of the patients exhibited insulin independence, 5 years later (Ryan et al. 2005). The limitations of survival were overcome, with the advent of novel T-cell depleting technologies, discovered recently (Bellin et al. 2008).
The deficient supply of cadaveric islets continues to hinder cell therapy for diabetes using human islets. Hence, the advent of clinical grade β-islets from stem cells is looked upon as a promising option for cell therapy to millions of patients, as opposed to hundreds in the case of cadaveric source.
13.4.2 Mesenchymal Stem Cells for Cell Therapy for Diabetes
Although mesodermal in origin, MSCs have the potential of multilineage differentiation (Pievani et al. 2014). The indicative markers for MSC are the presence of cluster of differentiation (CD) markers like CD73, CD105, and CD90 and an absence of hematopoietic stem cell markers like CD45, CD14, and CD34. MSCs can be isolated from various tissues like stroma of bone marrow, adipose tissue, and cord blood (Mosna et al. 2010).
Due to the easy availability of MSCs in large quantities, scientists have attempted to differentiate MSCs into functional insulin-producing β-cells, with varied degrees of success. Despite the multilineage potential of MSCs, mesodermal origin of MSCs makes them less efficient to differentiate into β-cells, of endodermal origin. Embryonic stem cells (ESC) can better differentiate into functional β-cells as compared to MSC (Jiang et al. 2007a, b; Kroon et al. 2008; Bose et al. 2012; Jeon et al. 2012). β-cell differentiation has been attempted from various types of MSC as bone marrow (Gabr et al. 2013; Karnieli et al. 2007), adipose tissue (Timper et al. 2006), umbilical cord blood (Prabakar et al. 2012), wharton jelly (Wu et al. 2009; Hu et al. 2013), dental pulp (Kanafi et al. 2013), placenta (Susman et al. 2010; Chang et al. 2007), and amnion mesenchymal stromal cells (Kadam et al. 2010a, b).
From a clinical viewpoint, postnatal MSCs like bone marrow, dental pulp, and adipose tissue MSCs can be used as autologous sources. Human extra-embryonic, tissue-derived peri-natal MSCs like amnion, placenta, Wharton jelly, and cord blood can be used as allogeneic sources to derive β-cells for transplantation (Bhonde et al. 2014). Although pluripotent stem cell-derived β-cells are easy to obtain, due to successful β-cell differentiation protocols, MSCs pose less or no ethical concerns, and no possibility of harmful effects of teratomas, unlike PSCs (Domínguez-Bendala et al. 2012; Davis et al. 2012).
In laboratory scale, all the aforementioned MSCs have been successfully differentiated into insulin-producing β-cells, which have also been tested on preclinical models of diabetes to ameliorate diabetes. Most importantly, autologous mesenchymal stem cells have been extremely successful, in clinics, from the safety point. In a case study 41 patients, all of whom received cell transplantation for cartilage repair of various joints, were followed for a period of up to 11 years and 5 months, with no adverse reactions (Wakitani et al. 2011).
To the best of our knowledge, there are no clinical trials which have used autologous bone marrow MSCs for replacing damaged β-cells. Interestingly, a recent report has harnessed the immunomodulatory property of MSCs to reduce the intensity of type I diabetes. MSCs injected through liver puncture in two patients resulted in the reduction of islet cell antibodies (ICA), glutamic acid decarboxylase (GAD), and insulin antibodies in 12 months, which, in turn, reduced the autoimmune destruction of islet cells in the patients (Mesples et al. 2013). Similarly, cord blood MSCs have also been harnessed, to improve the immune modulation and insulin resistance of type II diabetic patients, and are in phase I/II/clinical trials (Zhao et al. 2013).
13.4.3 Pluripotent Stem Cells for Cell Therapy for Diabetes
Although ample amount of success has taken place regarding the clinical transplantation of cadaveric islets into the liver, which could reverse diabetes (Halberstadt et al. 2013), the source of islets from the PSC source has yet to be in the clinics. Enhanced plasticity of PSCs has given greater hopes for the generation of β-cells. A large body of literature exists regarding differentiation of PSCs into β-cells. Various strategies/differentiation protocols for obtaining β-cells from PSCs were designed, primarily by mimicking in vivo pancreatic organogenesis (Jiang et al. 2007a, b; Kroon et al. 2008; Bose et al. 2012, 2014; Jeon et al. 2012).
Essentially they made use of various growth factors, in a stepwise manner, over the duration of 1 month or more. In addition to the growth factors, many protocols used small molecules for in vitro β-cell differentiation (Borowiak et al. 2009; Chen et al. 2009). The pitfalls are the presence of undifferentiated cell populations, and hence sorting for pure populations of differentiated β-cells or progenitor cells are a highly sought-after alternative (Fishman et al. 2012). Also, Fishman et al. successfully generated human ESC clones harboring GFP reporter constructs of Sox17, a definitive endoderm marker, and Pdx1, a pancreatic marker. Expression of GFP, in the ESC clones generated by Fishmann et al., helped obtaining pure populations of Sox17+/Pdx1+ pancreatic progenitor populations. The same strategy can be very useful for transplantation purposes. β-cells differentiated from human pluripotent stem cells (hPSCs) have been successfully tested on preclinical models of diabetes.
Per regulatory guidelines of most countries, any cell therapy product must be generated under good manufacturing practice (GMP) rules, in specifically licensed laboratories. Accordingly, the entire licensing process involves stringent quality control inspections and approval of raw materials, manufacturing, supply, and storage. In the case of hPSC-based cell therapy products, potential of tumorigenicity, inappropriate or incomplete differentiation, and potential of in vivo toxicities need to be evaluated on preclinical models before entering clinical trials. Also, in case of ESC therapy products, there are ethical concerns regarding the destruction of an embryo, while iPS cell therapy products are free from such ethical concerns.
Geron Corporation, a California-based company, received the first US FDA clearance on 2009, for a hESC-based cell therapy product, and initiated the first clinical trial. It was GRNOPC1, embryonic stem cell-derived oligodendrocyte progenitor cells, for treating patients with acute spinal cord injury. The trial was however discontinued in 2011, after treating four patients, because the company felt that the treatment was too expensive, and hence decided to channelize the funding for other projects (Lukovic et al. 2014).
Subsequently, Advanced Cell Technology entered into a Phase I/II trial, using GMP grade MA09 hESC line-derived retinal pigment epithelial (RPE) cells, for treating macular degeneration. Four months follow-up showed favorable results in two patients (Schwartz et al. 2012). Pluripotent stem cell-based clinical trials for diabetes have not yet been planned nor are they in the pipeline till date.
13.5 Details of Generation of Cell Therapy Products for Diabetes-Pluripotent Stem Cell Therapy: Mesenchymal Stem Cell Therapy
13.5.1 Details of Generation of Mesenchymal Stem Cell Therapy Products for Diabetes
Ethically acceptable with the possibilities of autologous source, generation of mesenchymal stem cell therapy products for diabetes should harness and maintain four basic properties of MSCs, such as (1) ability to migrate to the inflammation sites upon intravenous injections (2) ability to differentiate into functional β-islets (3) ability to secrete multiple bioactive molecules, which can inhibit inflammation, and heal the weak or dying β-cells (4) immunomodulatory functions of MSCs, along with lacking immunogenicity (Wang et al. 2012; Stagg and Galipeau 2013). Accordingly, large quantities of clinical grade MSCs can be generated under certified GMP conditions, from various sources like bone marrow, adipose tissue, and cord blood.
The scale-up of MSCs can be done under xeno free conditions. Hence, scaled up MSCs can directly be injected to the patients, in coherence with all the mentioned properties except for the second, which require additional steps of in vitro differentiation into functional β-islets, before transplantation. Furthermore, they can also be used as an adjuvant for better engraftment of transplanted β-islets from various sources like cadaveric and differentiated from human MSCs or PSCs (Rosengren et al. 2009).
Unaltered bone marrow MSCs injected into diabetic mice have also exhibited the potential of in vivo differentiation into functional β-islets (Iskovich et al. 2012). Hence, unaltered scaling up of human autologous bone marrow stem cells can be clinically useful, as they can differentiate in vivo into functional β-islets.
With regard to in vitro differentiation of β-islets prior to transplantation, the generation of cell therapy products requires a lot of culture manipulations. Such manipulations should follow various established protocols of β-islet differentiations from MSCs. It is important to scale-up the differentiation protocols by minimizing unwanted cell types, incomplete differentiations or differentiations, into possibly precancerous cells. In laboratory scale, protocols have a duration of approximately 2 months, and are spread over 2–3 steps. These involve 1,000-fold expansions of MSCs, transdifferentiation of MSCs first into endodermal cells, followed by β-cell precursors in culture. Alternatively, the MSC can directly be subjected to culture conditions to obtain transplantable functional β-cell precursors.
A specific example of human bone marrow MSC involves a 2 months 5 days differentiation protocol (Tang et al. 2012). In this three-step protocol, first step involves culturing of human BM-MSCs in RPMI-1640 media containing bFGF, the second step involves treatment of BM-MSCs with bFGF and EGF, and the third step involves obtaining transplantable β-cell precursors using 5.5 mM glucose and 5 % FCS (Tang et al. 2012).
In case of adipose tissue-derived mesenchymal stem cells (AD-MSC), pancreatic organogenesis is mimicked (Chandra et al. 2009). AD-MSC differentiation protocol involves the use of cytokines, inducing definitive endoderm and pancreatic progenitors like activin A, GLP-1, and nicotinamide, respectively, in a stepwise fashion (Chandra et al. 2009). Similarly, dental pulp-derived MSCs also involve steps mimicking in vivo pancreatic organogenesis, and make use of cytokines along with activin A, Na-butyrate, GLP-1, and nicotinamide (Govindasamy et al. 2011). Also, differentiation schemes of perinatal MSCs, from umbilical cord and Wharton jelly, involve mimicking in vivo pancreatic organogenesis, and make use of definitive endoderm and pancreatic endoderm inducing cytokines including activin A, nicotinamide, exendin, GLP-1, and Na-butyrate (Gao et al. 2008; Tsai et al. 2012).
The donors should qualify in the screening tests designed by the regulatory bodies (Sensebé et al. 2011). Such stringency holds good for allogeneic donors, where one donor serves for multiple patients, awaiting to receive MSC-based cell therapy products. Screening tests for MSCs donors are similar to donors of other cell therapy products, and primarily involve health questionnaire and viral testing. Age is another important criterion. BM-MSCs from child donors are considered to be of high quality, owing to a higher concentration of colony forming unit fibroblast precursors (CFU-Fs) than from adults (Baxter et al. 2004). Also, increasing age of the donor for BM-MSCs adversely affect the proliferation and multipotency of MSCs (Stolzing et al. 2008).
BM is obtained from the donor’s posterior superior iliac spine or crest, using an Illinois needle, or equivalent aspiration in a heparin-containing syringe (Bain 2001). Sample processing is done based on density gradient centrifugation, direct plating, or different enrichment strategies. Immunomagnetic beads-based enrichment of BM-MSCs are done on the basis of markers like STRO-1, CD49a, CD105, CD133, CD146, CD271, SSEA-4, antifebrin microbeads, aptamers, and aldehyde dehydrogenase activity (Guo et al. 2006; Gentry et al. 2007; Caplan 2007). However, the most common method to obtain clinical grade MSCs involve density gradient centrifugation of the bone marrow, followed by direct plating of the fractions on plastic cell culture dishes, to separate mesenchymal from hematopoietic cells.
A frequency of one MSC is obtained per million nucleated cells from adult bone marrow, while one MSC is obtained per 104 nucleated cells of umbilical cord blood (Gentry et al. 2007; Lu et al. 2006). Frequency of obtaining MSCs reportedly decreases tenfold from birth to teenage and a further tenfold from teenage to adulthood (Gentry et al. 2007; Lu et al. 2006; Caplan 2009). Similarly, high-quality MSCs are obtained from adipose tissues, using centrifugation to discard adipocytes, followed by cell plating and extensive washings (Zuk et al. 2001), as manual methods, and automated systems like Celuton system, Cytori Therapeutics, San Diego, CA (Duckers et al. 2006). Umbilical cord blood-derived MSCs are obtained by standard process of gravity assisted collection, by cannulating one of the umbilical veins after delivery of the placenta, under aseptic conditions, and processing the cord blood for obtaining MSC (Bieback et al. 2004).
Other important parameters for obtaining clinical grade MSCs are MSC expansion media like α MEM or DMEM (Sekiya et al. 2002; Sotiropoulou et al. 2006). Fetal bovine serum (FBS) is an important component for MSC proliferation (Caterson et al. 2002). However, addition of FBS reportedly induces immunogenic properties to MSCs, thereby rendering them clinically inferior (Sundin et al. 2007). Accordingly, non-FBS human-derived products like basic FGF, TGFβ1, IGF, and PLT lysates are the preferred alternatives for clinical grade MSCs (Bieback et al. 2009).
Hypoxic conditions reportedly cause reduced oxidative damage to the MSCs, thereby maintaining their multipotentiality (Berniakovich and Giorgio 2013). Plating density from low (1–5 × 103 per cm2) to very low (1–5 × 101/cm2) is also conducive for maintaining multipotentiality of MSCs and hence should be used for maintaining clinical grade MSCs (Banfi et al. 2000).
Large scale MSCs need to be manufactured in cGMP compliant bioreactors (Chen et al. 2006; Gastens et al. 2007). Storage/cryopreservation comprises 10 % DMSO with electrolyte solution (plasmalyte A), and a protein source (human serum albumin), and freezing at the rate of 1 °C per minute. Recommended transport are dry shippers or liquid nitrogen (Hanna and Hubel 2009). Most important, all clinical grade MSCs should be tested for endotoxins, mycoplasma (Munson 1985), as well as functional potential like trilineage differentiation potential (Kim et al. 2009), immunomodulation (Bifari et al. 2008), and replicative senescence (Wagner et al. 2008).
13.5.2 Details of Generation of Pluripotent Stem Cell Therapy Products for Diabetes
Pluripotent stem cell-based therapies are one of the most promising areas of medicine, and many such therapies are now being developed by industrial and academic groups. Cell therapies based on adult stem cells have been in use for several decades. First successful bone marrow transplant was carried out in 1968 (Bach et al. 1968; De Koning et al. 1969). Although there are PSC products for diabetes in laboratory scale, none of the PSC-based products are in clinical trials. The general strategy for adult and all PSC therapies is the scale-up of undifferentiated cells, differentiation to a specific cell type, and delivery to the patient. Some of the regulatory issues surrounding this strategy arise from the fundamental characteristics of PSC. Cells change over time both in vitro and in vivo, integrate and migrate after transplantation, and interact with host immune systems.
Cell products derived from PSCs present additional issues, like extensive replicative capacity and pluripotency. An advantage of PSC over MSC is the ability of PSC to replicate infinitely, in contrast to MSC, which has limited replicative ability, and thus gives rise to large quantities of cell yield. Such large quantities of cell yield from PSCs are advantageous for commercial cell therapy products. However, the ability of the cells to proliferate indefinitely will require the assessment of stability of the cells over time in vitro.
The pluripotency of the cells allows generation of a wide array of differentiated cell products, but also entails the possibility of teratoma formation, and the presence of unwanted cell types. For the safety of hESC/hiPSC (hPSC)-based cell therapies, the cells will require evaluation of the starting material, demonstration of a reproducible differentiation and cell processing, assessment of the identity of the final cell product, and characterization of in vivo properties, such as bio-distribution, tumorigenicity, toxicity, and immunogenicity.
Over the past decade, islet transplantation trials have demonstrated that patients with type I diabetes can be temporarily cured, by replenishing β cells and eliminating their dependency on insulin. The success of these trials is a proof that cell replacement therapy is a good option for treating diabetes. Due to scarcity or lack of donor islets, efforts are ongoing from many investigators in search for an alternative source of β cells, from human embryonic (hESCs) and iPSCs. Over the past few years significant research has been done in generating β-islets from hPSCs and as a result, it is now possible to generate insulin-producing cells from both hESCs and iPSCs.
Detailed steps of hPSC differentiation protocols always mimic in vivo pancreatic organogenesis. D’Amour et al. (2005) took a stepwise approach to set out to first differentiate hESCs toward definitive endoderm, a prerequisite for all pancreatic cell types. Also Shi et al. (2005) demonstrated the same approach to first differentiate the PSCs into definitive endoderm, followed by the differentiation into pancreatic β-islet cells, through the use of various growth factors in mouse cells. Later on, D’Amour et al. (2006) described a five-step and a four-step two-dimensional (or monolayer) hESC differentiation protocol that leads to efficient Pdx1 activation by day 12 and 15, respectively, and the impressive formation of up to 12 % insulin-containing cells.
Other protocols, which also mimicked in vivo pancreatic development, resulted in the formation of transplantable functional β-islet cells by day 18 (Madsen and Serup 2006) and day 25 (Jiang et al. 2007a, b).
The use of different model systems to understand early embryonic development had shown that nodal, a soluble molecule of the TGFβ/activin signaling family, was required for mesoderm and definitive endoderm formation during gastrulation. Higher levels of nodal were reported to promote endoderm specification (Conlon et al. 1994; Osada and Wright 1999; Lowe et al. 2001; Vincent et al. 2003). Based on these observations, D’Amour and colleagues implemented a protocol, in which hESCs were exposed to distinct soluble signaling factors. Activin A, another member of the TGF-β signaling family, was used together with Wnt3a to generate cells expressing markers of definitive endoderm (D’Amour et al. 2005, 2006).
The approach of using embryonic signals to instruct hESCs to definitive endoderm, and subsequently to β-cells, was validated by several other groups using modifications of this procedure (Jiang et al. 2007a, b; Eshpeter et al. 2008; Mao et al. 2009). These cells generated by D’Amour et al. (2006) responded to a variety of insulin secretagogues, but only showed limited glucose responsiveness. Modified pancreatic differentiation by Kroon et al. (2008) resulted in insulin-producing cells from hESC, which were also in vivo responsive to glucose. More recently, Nostro et al. (2011) reported improved efficiency (25 %) of insulin-producing cells by stage-specific in vitro regulation of TGF-β family members.
Specificities of in vitro differentiation protocols from PSC are ensured by detailed studies of pancreatic organogenesis. For example, within the mouse embryonic foregut, pancreatic fate is first specified by the expression of the homeobox gene Pdx1 (pancreatic and duodenal homeobox-1) (Jonsson et al. 1994; Guz et al. 1995). Thus, activation of Pdx1 is considered a prerequisite for pancreatic differentiation in vitro, and should precede the progressive expression of more mature markers of the endocrine lineage, including Ngn3, Nkx2.2, Nkx6.1, MafA, and MafB (Jensen 2004). Moreover, MafA has been identified as the master regulator of glucose-stimulated insulin secretion, in functional β-islet cells (Hang and Stein 2011; Matsuoka et al. 2004; Zhang et al. 2005; Wang et al. 2007).
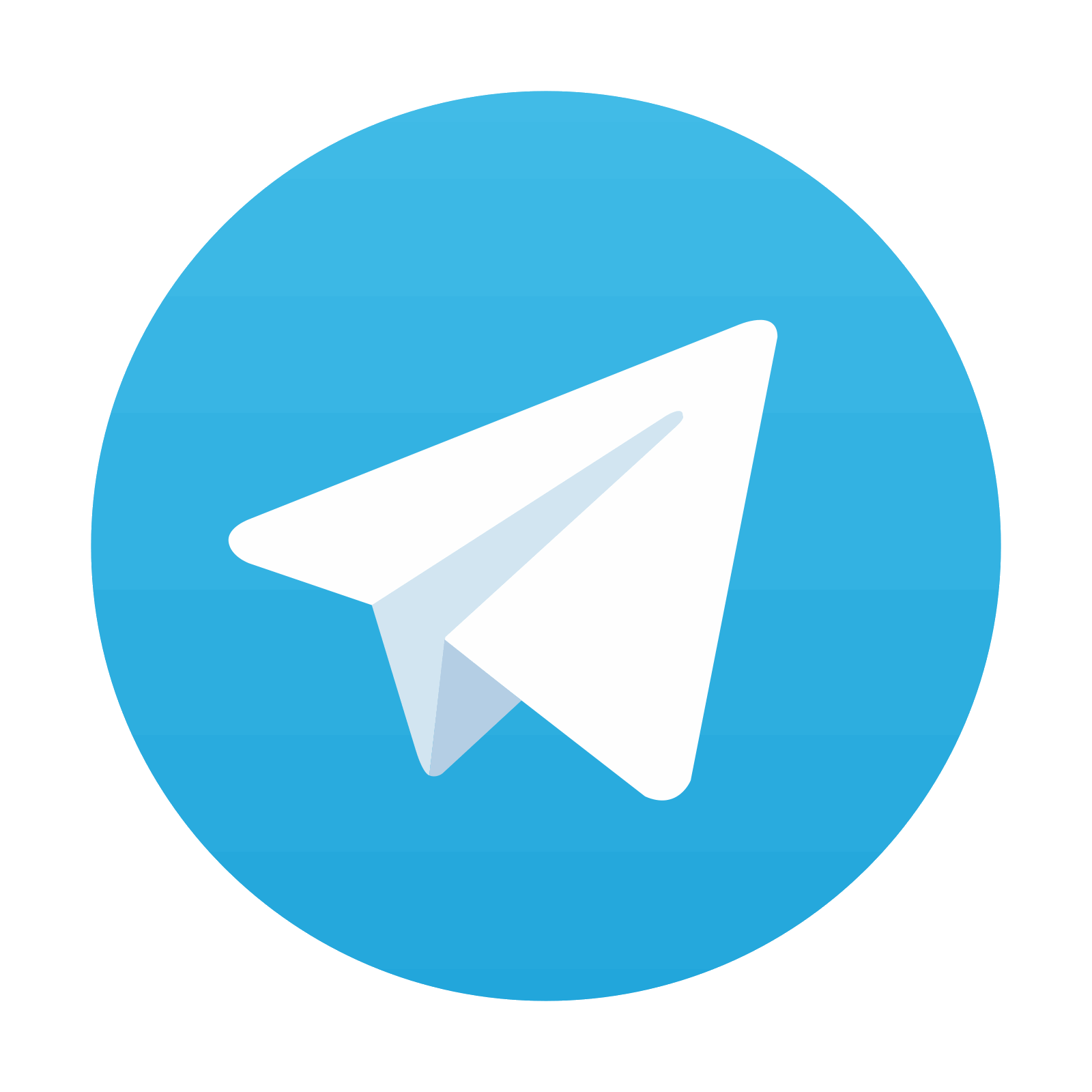
Stay updated, free articles. Join our Telegram channel
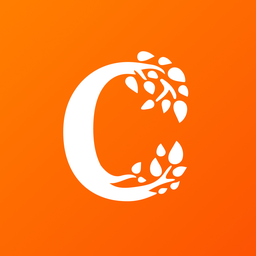
Full access? Get Clinical Tree
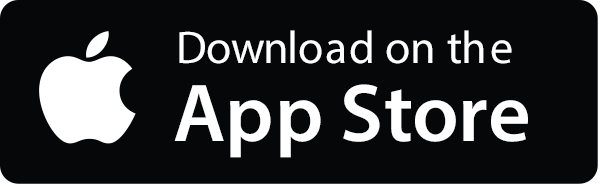
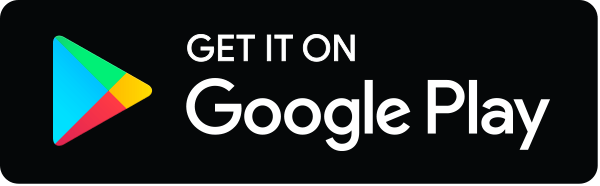