© Springer International Publishing AG 2018
Laurence Zitvogel and Guido Kroemer (eds.)Oncoimmunologyhttps://doi.org/10.1007/978-3-319-62431-0_33. CD8+ T Cells in Immunotherapy, Radiotherapy, and Chemotherapy
(1)
Department of Surgery, University of Michigan School of Medicine, Ann Arbor, MI 48109, USA
(2)
Department of Obstetrics and Gynecology, University of Michigan School of Medicine, Ann Arbor, MI 48109, USA
(3)
Department of Radiation Oncology, University of Michigan School of Medicine, Ann Arbor, MI 48109, USA
(4)
Graduate Programs in Immunology and Tumor Biology, University of Michigan, Ann Arbor, MI 48109, USA
Keywords
CD8+ T cellTumor immunityImmunotherapyCheckpoint blockadePD-L1PD-1ChemotherapyRadiotherapy3.1 Introduction
Extensive studies have revealed that the infiltration of T cells, especially CD8+ T cells, into the tumor microenvironment is a favorable prognostic feature for numerous malignancies, including melanoma and head and neck, breast, ovarian, renal, bladder, urothelial, colorectal, prostatic, pancreatic, and lung cancers [1]. A high density of intratumor CD8+ T cells is associated with longer disease-free survival and overall survival. Interestingly, direct tumor contact by CD8+ T cells may not be required, as both intraepithelial and intrastromal CD8+ T cells are associated with a favorable prognosis in human breast and ovarian cancer [2–4].
CD8+ T cells are often referred to as cytotoxic T lymphocytes (CTLs) because of their ability to directly kill target cells. Upon antigenic stimulation, CD8+ T cells will progressively differentiate from naïve T cells into central memory T cells (TCM) and effector memory T cells (TEM). The effector function increases upon CD8+ T-cell differentiation, while memory function and proliferation decrease. Effector CD8+ T cells are characterized as CCR7− CD62L−CD45RO+CD95+IL-2Rb+, in addition to expressing killer cell lectin-like receptor G1 (KLRG-1) and programmed death 1 receptor (PD-1). They secrete high concentrations of IFNγ and TNFα but secrete minimal IL2. Trafficking of CD8+ T cells is mediated through chemokine-chemokine receptor interaction, which, among others, includes the ligands CXCL9 and CXCL10 with their receptor CXCR3. Increased expression of CXCL9/10 is associated with increased number of CD8 T cells in tumor microenvironment [5, 6].
CD8+ T cells are a key component of antitumor immunity and execute tumor clearance by several mechanisms. First, CD8+ T cells recognize the specific tumor-associated antigen [7] expressed on tumor cells, release cytotoxic molecular granzyme B and perforin, which are delivered into tumor cells and induce caspase activation and ultimately apoptosis [8]. Secondly, CD8+ T cells can also induce cancer cell death through the Fas/Fas ligand pathway. It has been demonstrated that the Fas ligand is essential for tumor regression mediated by CD8+ T cells in murine models of lung cancer and B-cell lymphoma [9, 10]. Finally, IFNγ and TNFα secreted by CD8+ T cells can have antitumor activity and control tumor growth. The combination of IFNγ and TNFα can drive cancer cell into senescence [11]. IFNγ is also known to be critical for cancer immunosurveillance by enhancing antigen presentation and limiting tumor angiogenesis [12].
Although tumor-reactive CD8 T cells are often found in the tumor biopsies, cancer can still progress. It has been revealed that the immunosuppressive tumor microenvironment may drive CD8 T cells into senescence or exhaustion [13]. Senescent CD8+ T cells are characterized by short telomeres, activation of DNA damage response genes, and secretion of senescence-associated secretory phenotype (SASP) factors [14]. These cells phenotypically show downregulation of the co-stimulatory molecules CD27 and CD28 and high expression of CD57 and KLRG1. Although senescent T cells are irreversibly cell-cycle arrested, they may still retain their cytotoxic capacity [15]. Exhausted CD8+ T cells are described as cells that exhibit defects in proliferation and decreased cytokine production and cytotoxic functions, as well as display higher expression of co-inhibitory molecules, such as PD-1, CD244, CD160, CTLA-4, Lag-3, and Tim-3 [16, 17]. However, it is notable that CD8+ T cell exhaustion is reversible to some extent [18]. Blockade of CTLA-4 or PD-1 has been shown to improve CD8+ T-cell effector function, resulting in improved clinical response. In addition, adoptive transfusion of ex vivo-expanded tumor-specific T cells, especially CD8+ T cells, has achieved durable tumor remission and even cure of malignant disease. Interestingly, recent studies have revealed that antibody-based targeted therapy, radiotherapy, and chemotherapy may synergistically initiate or augment antitumor immune response. The antitumor efficacies of these therapies are at least partially dependent on CD8+ T-cell immunity.
This review focuses on the convergence of adoptive T-cell transfer, checkpoint blockade, antibody-based targeted therapy, radiotherapy, and cytotoxic chemotherapy on effector CD8+ T cells. We summarize the state of knowledge regarding how these therapies increase intratumor CD8+ T-cell infiltration, induce tumor antigen-specific CD8+ T-cell response, unleash CD8+ T-cell effector function, and sensitize tumor to CD8+ T cells. Finally, we discuss how to rationally combine immunotherapy with radiotherapy or/and chemotherapy to improve cancer patient outcomes.
3.2 CD8+ T Cells in Immunotherapy
3.2.1 Adoptive T-Cell Transfer
Adoptive T-cell therapy (ACT) for cancer is a form of transfusion therapy consisting of the infusion of various ex vivo-expanded T-cell populations. The first strategy of ACT, which has been the most extensively studied in clinical trials, is the adoptive transfer of autologous ex vivo-expanded tumor-infiltrating lymphocytes (TILs). More recently, transfer of genetically modified T cells is being developed and clinically utilized. This approach includes the utilization of peripheral blood lymphocytes (PBLs)-derived T cells expressing TAA-specific T-cell receptor (TCR) or a so-called “chimeric antigen receptor” (CAR) T cells [19, 20].
CD8+ T in Tumor-Infiltrating Lymphocytes Therapy
The general protocol of ACT includes (1) collection of circulating or tumor-infiltrating lymphocytes, (2) selection and expansion of tumor-specific T-cell populations ex vivo, and (3) re-administration of T cells to patients with a conditioning regimen of lymphodepletion and IL-2 administration. Thus far, ACT of ex vivo-expanded TILs is considered to be the best available treatment for patients with chemorefractory metastatic melanoma [21, 22]. Following the harvesting of TILs from the patient, long-term ex vivo IL-2 and CD3 stimulation are used to expand CD4+ and CD8+ αβ TCR+ T cells [21]. As CD8 interacts with MHC class I expressed on tumor cells, CD8+ T cells are thought to effectuate the antitumor activity of ACT, although indirect CD4+ T-cell interaction with the tumor cannot be dismissed. A recent clinical study described three sequential trials on metastatic melanoma treated with the ACT of autologous TILs combined with lymphodepletion and IL-2. Objective response rates in the three trials using different lymphodepleting preparative regimens ranged from 47% to 72% [22]. Furthermore, the number of infused CD27+ CD8+ cells was found to correlate with objective response [22]. This corroborates other melanoma ACT trials that have also found a positive correlation between a higher number of infused CD8+ T cells and clinical response [21, 23].
Nonselective expansion of polyclonal tumor-infiltrating T cells results in a population that recognizes multiple tumor-associated antigens. These antigens include cancer testis antigens that are expressed during development and reactivated in tumors, such as NY-ESO-1 and MAGE; melanocyte lineage antigens, such as gp100, MART-1, and tyrosinase; and mutational antigens generated from the low-fidelity replication present in cancer cells. A recent study analyzed the antigens recognized by clinically effective TILs from melanoma patients that experienced durable complete regressions beyond 5 years after ACT of TILs and identified both nonmutated and mutated antigens that could be recognized by autologous TILs [24]. More recently, neoantigen-specific T cells including CD8+ T cells were successfully isolated from the blood and primary tumor in patients with melanoma [25]. A recent case report demonstrated that ACT from TILs in a patient with metastatic KRAS mutant colorectal cancer could result in durable regression of all metastatic deposits. Correlative studies revealed that four different CD8+ T-cell clones that were specifically reactive to mutant KRAS G12D mediated this response [26]. This highlights an emerging strategy where cellular immunity can be harnessed to target conserved oncogenetic mutations, which have not been conducive to pharmacologic inhibition.
In addition to TAA specificity, emerging findings indicate that the differentiation state of T-cell populations is crucial to the antitumor efficacy of ACT [20, 27]. CD8+ T cells in ex vivo-expanded TILs are a mixture of mostly TEM (less-differentiated effector memory T cells), TEFF (more-differentiated effector T cells), and TTDE (terminally differentiated effector T cells). Relatively, very few TCM (central memory) are found in the ex vivo-cultured TILs, although in preclinical and clinical models TCM cells have shown increased antitumor activity compared with effector T cells in mouse melanoma models [28, 29]. Currently, little is known on which state of differentiated CD8+ T cells is optimal for ACT of TILs in human.
Genetically Engineered T Cells
T cells can be genetically engineered to express a T-cell receptor (TCR) with a high affinity and specificity to target antigens. Introductions of such TCR genes are accomplished by retrovirus or lentivirus-mediated transduction. Such TCR-modified T cells have specificity for tumor-associated antigens and can be rapidly expanded ex vivo and reinfused into patients for ACT. For example, TCR transduction has been used to target MART-1 and NY-ESO-1 in clinical trials for patients with melanoma. Tumor regression and durable objective responses were observed in a subset of patients [30, 31].
Chimeric antigen receptors (CARs) are another means for providing specificity to transduced T cells. CAR molecule is an artificial receptor composed of a single-chain variable fragment (scFv) derived from antibody, fused to transmembrane and cytoplasmic domains. The scFv fragment recognizes specific surface tumor antigens in an MHC-independent fashion. The cytoplasmic domains consist of a CD3 zeta activation domain and two co-stimulatory domains, CD28 and CD137/4-1BB. Upon antigen encounter, the CAR transduces the activation signals to T cells, resulting in T-cell proliferation and expansion with cytotoxic functions [32]. Clinical trials have shown excellent outcomes for CAR-T-cell adoptive transfer therapy in patients with hematologic malignancies [33]. Almost all B-cell malignancies, as well as normal B cells, express the CD19, which is absent in other cell types. Thus, anti-CD19-redirected CAR-T cells were designed to target CD19+ B cells and have achieved impressive response rates in 60–90% of patients with relapsed or refractory lymphoblastic leukemia [34–36]. CAR-T cell therapies are also being developed to target solid tumors in a number of disease sites [37, 38]; however, these efforts have been historically hindered by off-target toxicity [39, 40].
In a manner similar to TILs, genetically modified T cells for ACT also contains both CD4+ and CD8+ T-cell populations, which both confer an antitumor response. In a recent clinical trial on patients with B-cell malignances, CD19-CAR-T cells were generated from CD8+ and CD4 T-cell subsets that were separately ex vivo expanded and infused at a 1:1 ratio. This defined composition product showed remarkable antitumor activity as 93% patient achieved bone marrow remission [41]. In contrast to polyclonal TILs, genetically modified T cells have monoclonal specificity to a single target antigen, which may facilitate tumor immunoediting and allow the development and outgrowth of antigen escape tumor subclones.
3.2.2 Checkpoint Blockade
The immune system is characterized by compensatory inhibitory mechanisms to prevent the inflammatory response from precipitating autoimmunity. Tumor-infiltrating T cells that recognize and are poised to eliminate tumor cells are held in check by negative signals that reduce their activation and effector functions. Several molecules have been identified as negative regulators or checkpoints of T-cell activation, including cytotoxic T-lymphocyte-associated antigen 4 (CTLA4), PD-1, and PD-L1. Drugs interrupting these checkpoints can unleash the antitumor activity of T cells and mediate durable cancer regressions. Multiple therapeutic antibodies that block CTLA4, PD-1, or PD-L1 have been approved and have shown clinical benefits in a wide range of solid and liquid tumor types, including melanoma, non-small cell lung cancer, kidney cancer, and Hodgkin’s lymphoma [42].
The Biology of the CTLA-4 Pathway
CTLA-4 is a receptor that is expressed exclusively on T cells and primarily regulates the amplitude of the early stages of T-cell activation. The engagement of CTLA-4 downregulates the T-cell function, largely by counteracting the activity of the T-cell co-stimulatory receptor, CD28. The recognition of peptide-major histocompatibility complex (MHC) by the T-cell receptor (TCR) is insufficient for T-cell activation and must be amplified by the ligation of CD28 to its ligands, CD80 and CD86. CTLA-4 shares the same set of ligands with CD28 but with a much higher affinity; therefore, its expression on the surface of T cells dampens the activation of T cells by outcompeting CD28 with regard to binding CD80 and CD86, as well as actively delivering inhibitory signals to the T cell [43]. CTLA-4 also confers T-cell inhibition via depletion of CD80 and CD86 from the antigen-presenting cell (APC) surface [44]. The essential role of CTLA-4 for maintaining normal immunologic homeostasis is demonstrated by the lethal systemic immune hyperactivation phenotype in CTLA-4-deficient mice [45, 46].
On the basis of CTLA-4 biology, ipilimumab, a therapeutic antibody against CTLA-4, has been developed and approved for the treatment of patients with advanced melanoma. Ipilimumab binds to CTLA-4 and blocks ligation with CD80 and CD86, which prevents inhibitory signal transduction and results in increased CD28-mediated co-stimulation. CTLA-4 is predominantly expressed on CD4+ T cells, and CTLA-4 blockade has been demonstrated to mediate antitumor immune response through enhancement of effector CD4+ T-cell activity, as well as inhibition of regulatory T (Treg)-cell-dependent immunosuppressive activity. In Treg cells, CTLA-4 is regulated by the forkhead transcription factor FOXP3 and therefore constitutively expressed. It has been demonstrated that anti-CTLA-4 antibody can deplete Treg population in the tumor microenvironment in a Fc-mediated manner through antibody-dependent cellular cytotoxicity (ADCC) [47].
CD8+ T Cells in CTLA-4 Blockade
In addition to CD4+ T cells, CTLA-4 blockade enhances CD8+ T-cell response in the tumor microenvironment. Because CTLA-4 is also expressed on activated CD8+ effector T cells, CTLA-4 blockade is considered to directly regulate CD8+ T-cell activity. CTLA-4 regulates effector functions of CD8+ T cells through repressing the production of IFNγ and eomesodermin in individual CD8+ T cells [48, 49]. CTLA-4 blockade was shown to directly enhance the proliferation and activation of specific CD8+ T cells in vitro and in vivo, in a manner independent of CD4+ T-cell help [50]. However, studies using different mouse tumor models demonstrated that CTLA-4 blockade could also reverse CD8+ T-cell tolerance and mediated antitumor immune response by a CD4+ T cell-dependent mechanism [51, 52]. Regardless of which cell types are targeted by CTLA-4 blockade, the functional result of CTLA-4 blockade therapy is enhancement of tumor-specific CD8+ T cells and tumor regression. Ipilimumab treatment in melanoma patient results in clonal expansion of tumor-specific CD8+ T cells in the tumor microenvironment and systemic circulation, although it is related with ipilimumab-induced toxicities [53]. Ipilimumab also increases the absolute number of circulating CD8+ T cells, which correlated with improved clinical outcomes [54].
The Biology of PD-L1/PD-1 Pathway
PD-1 is a cell surface receptor of the same immunoglobulin family as CD28 and CTLA-4. Similar to CTLA-4, PD-1 is absent on resting naive and memory T cells and is induced after T-cell activation. However, in contrast to CTLA-4, PD-1 expression on the surface of activated T cells is initiated at a transcriptional level and is therefore delayed [55]. Unlike CTLA-4, which primarily regulates T-cell activation at the earlier stage, PD-1 is believed to inhibit effector T-cell activity in the effector phase within peripheral tissue and tumors [56]. Ligand engagement of PD-1 results in activation of the inhibitory phosphatases SHP-2 and PP2A, which suppress the kinase signaling required for T-cell activation [55, 57].
The ligands for PD-1 are PD ligand 1 (PD-L1, B7-H1, CD274) and PD ligand 2 (PD-L2, B7-DC, CD273) [58, 59]. PD-L1 has immunomodulatory functions independent of PD-1 and can also bind CD80 on activated T cells and APCs to deliver inhibitory signals [60, 61]. The relevance of this interaction in antitumor immune resistance has yet to be determined. Additionally, PD-L1 engagement results in bidirectional signaling that “back” transmits signals into T cells and tumor cells to regulate their survival [62, 63]. Thus, PD-L1 could regulate tumor immunity by functioning as both a ligand and receptor. Similarly, PD-L2 can deliver suppressive signals through PD-1 and can also signal via repulsive guidance molecule b (RGMb) to promote respiratory tolerance [64]. The relevance of PD-L2 signaling to cancer immunity is unknown as it is not widely expressed by tumor or immune cells.
Clinically, the PD pathway blockade, including anti-PD-1 and anti-PD-L1 antibodies, has demonstrated highly durable response rates with minimal toxicity across a spectrum of different tumor types, spanning both solid tumors and hematologic malignancies [65]. In theory, targeting PD-1 may result in different biologic effects than targeting PD-L1 because of the different cellular populations that express these two molecules. In addition to activated T cells, PD-1 expression was found on B cells and natural killer (NK) cells, and, therefore, PD-1 blockade may influence the function of these cells as well [66, 67]. PD-L1 was highly expressed on tumor cells and tumor-associated APCs, including dendritic cells (DCs), macrophages, fibroblasts, and T cells [68–72]. PD-L1 on different types of cells may mediate immunoregulation through unique mechanisms. The comparative effectiveness between anti-PD-1 and anti-PD-L1 antibodies cannot yet be performed because of clinical data that has not yet matured, and biological inferences from the clinical studies may be limited by the differing degrees of chimerism and different isotype subgroups of the antibodies.
CD8+ T Cells in PD Blockade
Although the cellular and molecular mechanisms are not completely defined, translational and clinical studies suggest that both PD-1 and PD-L1 blockades converge on tumor-infiltrating CD8+ T cells. In the tumor microenvironment, PD-1 is highly expressed on infiltrating lymphocytes, including tumor-specific CD8+ T cells, and engagement by PD-L1 on tumor cells or APCs results in CD8+ T-cell dysfunction. Analysis of melanoma patients treated with anti-PD-1 antibody (pembrolizumab) showed that the expansion of intratumoral CD8+ memory T cells was marked in those patients who responded to therapy [73, 74]. PD-1 blockade could enhance the proliferation of the effector memory CD8+ T cells with senescent phenotype [75]. Additionally, PD-L1 blockade was shown to reverse exhausted CD8+ T-cell function, and this could be synergized by anti-CD27 [76]. These studies suggest that both anti-PD-1 and anti-PD-L1 antibodies can enhance CD8+ T-cell proliferation and improve effector cytokine production to promote antitumor activity. Recent clinical studies on melanoma have further demonstrated that “inflamed” or “hot” tumors are highly responsive to PD pathway blockade [77]. An “inflamed” tumor is characterized by a Th1-type immune signature that includes Th1-type chemokines, CD8+ T cells, and a high level of PD-L1 expression [6, 65]. Tumor regression mediated by therapeutic PD blockade requires preexisting CD8+ T cells in the tumor microenvironment [73].
3.2.3 Antibody-Based Targeted Therapy
Antibody-based therapy for cancer has been established for more than a decade. The fundamental basis for this therapy is the differential upregulation or mutation of cell surface antigens on cancer cells, compared to normal tissues. Receptor tyrosine kinases, such as EGFR and HER2 (ERBB2), have been found to be overexpressed or mutated in various cancer types, including breast, lung, brain, head and neck, and colon tumors. Aberrant tyrosine kinase activity of EGFR and HER2 can promote cancer cell proliferation and tumorigenesis [78, 79]. Monoclonal antibodies targeting HER2 and EGFR have been approved by the FDA and are currently being utilized in a variety of disease sites [80]. These antibodies antagonize these oncogenic receptors, leading to reduced proliferation and increased apoptosis [78]. Additionally, the antitumoral effect of these antibodies is also mediated by the Fc region of antibody, which can bind to Fc receptors (FcRs) on macrophages, neutrophils, and natural killer (NK) cells and induce cell death through activation of complement-dependent cytotoxicity (CDC) and ADCC [80, 81].
Interestingly, recent studies suggested that adaptive immunity, including CD8+ T cells response, contributes to the efficacy of anti-HER2 and anti-EGFR antibodies. A murine HER2-overexpressing breast cancer model demonstrated that anti-HER2/neu antibody therapy required CD8+ T cells. Anti-HER2/neu antibody treatment increased CD8+ T-cell infiltration into tumor and induced memory T-cell responses [82]. This result was corroborated by another immunocompetent murine HER2 breast cancer model, which demonstrated that IFNγ-producing CD8+ T cells are required for efficacy of the antibody therapy [83]. Similarly, a study using a murine EGFR+ lung cancer model showed that anti-EGFR antibody cetuximab induced a tumor-specific CD8+ T-cell response, which is required for efficacy of antibody [84]. Additionally, cetuximab was shown to promote dendritic cell maturation and CD8+ T-cell priming, leading to the activation of tumor-specific T cells in patients with head and neck cancer [84, 85].
Bevacizumab, a humanized monoclonal antibody targeting vascular endothelial growth factor A (VEGF-A), is used in the treatment of many malignancies, including colon cancer, lung cancer, glioblastoma multiforme, and renal cell carcinoma. VEGF-A is a secreted factor that is critical for tumor angiogenesis through binding to the VEGFR1 and VEGFR2 receptors. Bevacizumab binds to and neutralizes all human VEGF-A isoforms and thereby block angiogenesis [86]. Aside from its direct action on tumor vascularization, anti-VEGF-A antibody has been shown to modulate immune cells in the tumor microenvironment. Blockade of VEGF-A increased DCs maturation and inhibited infiltration of immunosuppressive cells, such as regulatory T cells and MDSCs [87]. In a mouse model of colorectal cancer, VEGF-A was reported to regulate CD8+ T-cell exhaustion by enhancing expression of PD-1 and other inhibitory checkpoints, and this phenotype could be abrogated by an anti-VEGF-A antibody treatment [88]. VEGF-A can also inhibit the infiltration of T cells by reducing adhesion molecule expression in endothelial cells. Modulation or normalization of tumor vasculature by anti-VEGF-A antibody can result in increased T-cell recruitment and infiltration into tumors [89, 90]. In patients with metastatic renal cell carcinoma, bevacizumab therapy increased intratumoral CD4+ and CD8+ T-cell infiltration [91]. Increased intratumoral T cells were also observed in a combination therapy of bevacizumab with anti-PD-L1 antibody in renal cell carcinoma [92].
3.3 CD8+ T Cells in Radiotherapy
3.3.1 Radiation Therapy Induces Immune Responses
Radiotherapy is a highly effective treatment modality used for the curative and palliative management of almost all cancer histologies. It is frequently combined with other treatment modalities, including surgery, chemotherapy, and more recently immunotherapy, to maximize the chance of disease control [93]. Radiotherapy is a noninvasive localized therapy that applies ionizing radiation (IR) to a tumor. This induces single- and double-stranded DNA breaks in the irradiated tissue. As cancer cells are more sensitive to DNA damage-induced cell death than normal cells because of deficiencies in DNA repair pathways, ionizing radiotherapy can selectively damage cancerous cells [94].
Consistent with this, classical radiobiologic models have shown that radiotherapy induces tumor cell intrinsic mitotic catastrophe and cell death [95]. However, recent studies have highlighted the cell extrinsic mechanisms through which radiation modulates local or systemic immune responses and highlight the challenges and promise of combining radiotherapy with immunotherapy. Low-dose total body radiotherapy was used prior to hematopoietic stem cell transplant to create an immunosuppressed host with stem cell niche availability [96]. The hematopoietic compartment, which is comprised of hematopoietic stem cells, progenitor cells, and the vast majority of innate and adaptive immune cells, is vulnerable to radiation due to a rapid cycling time. Thus, even low doses of radiation are sufficient to induce cell death and damage in mature NK cells, T and B cells, as well as bone marrow stem cell precursors of monocytes and granulocytes. Low-dose radiotherapy was also historically used for the management of benign inflammatory conditions with moderate efficacy [97]. Finally, fractionated courses of radiotherapy had often been delivered in which small doses of radiotherapy are delivered on consecutive days for a duration up to 7 weeks to allow normal tissue healing and minimize treatment-associated toxicity. When fractionated radiotherapy is given to a large area with concurrent chemotherapy, incidental lymphopenia can result for several months, which can compromise ongoing efforts to promote tumor immunity [98].
In contrast to low-dose total body irradiation, emerging evidence demonstrates that high-dose localized radiation often initiates or enhances antitumor immune response, and the efficacy of radiotherapy even relies, in part, on the host innate and adaptive immunity [99, 100]. Over the last decade, advances in diagnostic imaging and radiotherapy delivery allow for more conformal treatments to a smaller volume without compromising local control. Radiotherapy techniques, including intensity-modulated radiotherapy, have been shown to decrease the toxicity of treatment at many disease sites, including the risk of lymphopenia [95]. Further, hypofractionated approaches, including stereotactic body radiotherapy and stereotactic radiosurgery, which provide equivalent or superior outcomes in one to five total treatments, are increasingly utilized in a variety of disease sites. Biologically, radiation induces immunogenic cell death by causing the release of tumor antigens and danger-associated molecular patterns (DAMPs), such as calreticulin, ATP, and high-mobility group protein B1 (HMGB1). DAMPs are endogenous molecules that induce immunostimulatory effects upon release or exposure during cell death and act by binding to pattern recognition receptors (PRR) expressed on innate immune cells. Simultaneously, radiation can create an inflammatory microenvironment by the induction of cytokine and chemokine production, which leads to infiltration of DCs, macrophages, cytotoxic T cells, and some immunosuppressive cells. Released DAMPs work on APCs through TLR4 signaling to promote efficient processing and cross-presentation of tumor antigens [101]. Mature APCs can migrate to the draining lymph node, where T-cell priming is augmented to initiate a systemic antitumor immune response.
3.3.2 CD8+ T Cells in Radiotherapy
Emerging evidences have demonstrated that radiotherapy can induce tumor-specific CD8+ T cell responses that are critical for radiation-mediated tumor reduction. Using a mouse B16 melanoma model, Lee et al. showed that ablative hypofractionated radiation induces significant tumor regression dependent on CD8+ T-cell activation and recruitment [102]. Radiation has also been shown to induce activation of tumor-associated DCs that support tumor-specific effector CD8+ T cells. The efficacy of radiotherapy depends on DCs and CD8+ T cells, whereas CD4+ T cells or macrophages are dispensable [103, 104]. More recent study suggested that CD8+ T cells and IFNγ contributed to radiation-induced tumor equilibrium in two animal models. Depletion of CD8+ T cells or neutralization of IFNγ leads to tumor regrowth, and blockade of PD-L1 augments CD8+ T-cell response and leads to tumor rejection [105].
Concomitant with increased T-cell activation, radiotherapy can diversify the TCR repertoire of tumor-infiltrating CD8+ T cells. Radiation increases the expression of MHC class I and the production of novel proteins to favor neoantigen presentation [106]. Another study has shown that radiation increases the expression of cancer testis antigens, which promotes the immunological recognition of cancer cells by T cells [107]. In a more recent study involving melanoma patients and a mouse melanoma model, TCR sequencing revealed that high-dose radiation increased diversity of TCR clonotypes of CD8+ TILs. The optimal antitumor response was achieved by the combination of the three treatment modalities: high-dose radiation, CTLA-4 blockade, and PD-L1 blockade [108].
Radiation could also promote tumor infiltration of CD8+ T cells through alterations in tumor vascularity and improved T-cell homing. Radiation induces a pro-inflammatory milieu including inductions of IFNγ as well as many other cytokines and chemokines. This leads to the infiltration of different immune cell subsets, including CD8+ T cells. Radiation-induced chemokines include CXC-motif chemokine 9 (CXCL9), CXCL10, CXCL11, and CXCL16, which binds to corresponding receptors on CD8+ effector T cells, resulting in migration of T cells into the tumor microenvironment. Moreover, type I IFNs were demonstrated to be required for the CXCL10 production within tumor after radiation treatment. Radiation-induced CXCL10 expression correlated with intratumor CD8+ T-cell numbers [109].
3.4 CD8+ T in Cytotoxic Chemotherapy
3.4.1 Chemotherapeutic Agents Activate Immune Responses
Cytotoxic chemotherapy is another efficacious treatment modality used for the management of many advanced cancers. Cytotoxic chemotherapy functions by inducing tumor cell death or inhibiting tumor cell reproduction. Based on their principal mechanism of action, conventional chemotherapeutic agents can be organized as several categories:
- 1.
Alkylating agents or DNA-damage agents, which cause DNA strand cross-link by adding alkyl groups to the electronegative groups and result in DNA-damage-induced cell death (e.g., cyclophosphamide and cisplatin)
- 2.
Antimetabolites, which function as the building blocks by imitating purine or pyrimidine to inhibit the synthesis of DNA and RNA (e.g., 5-fluorouracil)
- 3.
Spindle poisons, which interfere microtubule function and mitotic spindle assembly, resulting in cell-cycle arrest (e.g., paclitaxel and taxanes)
- 4.
Topoisomerase inhibitors, which prevent the correct unwinding of DNA during replication, transcription, and repair (e.g., irinotecan and etoposide)
- 5.
Antitumor antibiotics, which are made from natural products of soil fungus Streptomyces and exert antineoplastic effects by various mechanisms, including DNA intercalation, altering membrane fluidity, and generation of oxygen radicals (e.g., doxorubicin and bleomycin) [95]
The integration of cytotoxic chemotherapy with immunotherapy is the subject of several ongoing clinical trials.
Similar to radiotherapy, cytotoxic chemotherapy has historically been considered immunosuppressive because most chemotherapeutic agents indiscriminately impair cellular division and thus impact tumor cells, effector lymphocytes, and homeostasis of innate leukocytes [110, 111]. Cytotoxic chemotherapy is now the main backbone for conditioning regiments to generate lymphodepletion prior to HSCT and ACT [20]. However, recent studies demonstrated that select chemotherapy agents might also augment tumor immunity [112, 113]. Chemotherapy can initiate or promote antitumor immune response through two major mechanisms. First, chemotherapy induces immunogenic cell death on tumor cells [114]. Similar to radiotherapy, chemotherapy-induced ICD involves the release of tumor antigens and the emission of DAMPs in the tumor microenvironment. Immunogenic chemotherapy-associated DAMPs include calreticulin (CRT), heat shock protein HSP70 and HSP90, ATP, annexin A1, and HMGB1, although different drugs may correlate with different DAMPs [115–117]. For example, Obeid et al. reported that during anthracycline-induced cell death, CRT was exposed to the cellular surface and facilitates their engulfment by DCs, which leads to tumor antigen presentation and tumor-specific CTL response [115, 118]. The antitumor efficacy of many chemotherapy drugs has been demonstrated to partially rely on the induction of ICD. Secondly, chemotherapy agents could activate systemic immunity. Some chemotherapy drugs could directly stimulate the effector activity of myeloid or lymphoid cells. Paclitaxel was shown to promote DC maturation and cross-priming in mouse breast cancer model [119] and enhance the infiltration of NK cells in a cohort of breast cancer patients [120]. Cyclophosphamide has been shown to favor Th17 and Th1 memory response through altering the composition of microbiota in the small intestine [121]. Gemcitabine resorted defective cross-presentation of tumor antigen in DCs [122]. Finally, cytotoxic chemotherapy may also preferentially target immunosuppressive cells to indirectly enhance antitumor immune response. Gemcitabine [123], 5-FU [124], docetaxel [125], oxaliplatin [126], and paclitaxel [127] have all been shown to deplete blood-borne or tumor-infiltrating Treg cells or MDSCs.
3.4.2 Chemotherapy Enhances the Antitumor Function of CD8+ T Cells
Among innate or adoptive immune cells associated with chemotherapy, cytotoxic CD8+ T lymphocytes are considered a crucial mediator for tumor regression. The antitumor efficacies of these agents even rely in part on CD8+ T cells. In a mouse sarcoma model, the depletion of CD8+ T cells using anti-CD8+ antibody abolished anthracycline-mediated tumor regression, suggesting CD8+ T cells are indispensable for the anticancer efficacy of anthracyclines [128]. Using lung adenocarcinoma mouse models, the chemotherapy of oxaliplatin combined with cyclophosphamide was shown to induce antitumor response relied on innate immune sensing through TLR4 signaling and ultimately depended on CD8+ T-cell immunity [129]. Tumor regression induced by paclitaxel combined with blockade of IL-10 receptor was dependent on CD8+ T cells in a mouse model of orthotopic PyMT-derived tumors. Correlative studies in human breast cancer have found expression of CD8A to be predictive of pathological complete response [130] to neoadjuvant paclitaxel, and patients who achieve a pathological complete response (pCR) have improved clinical outcomes [131].
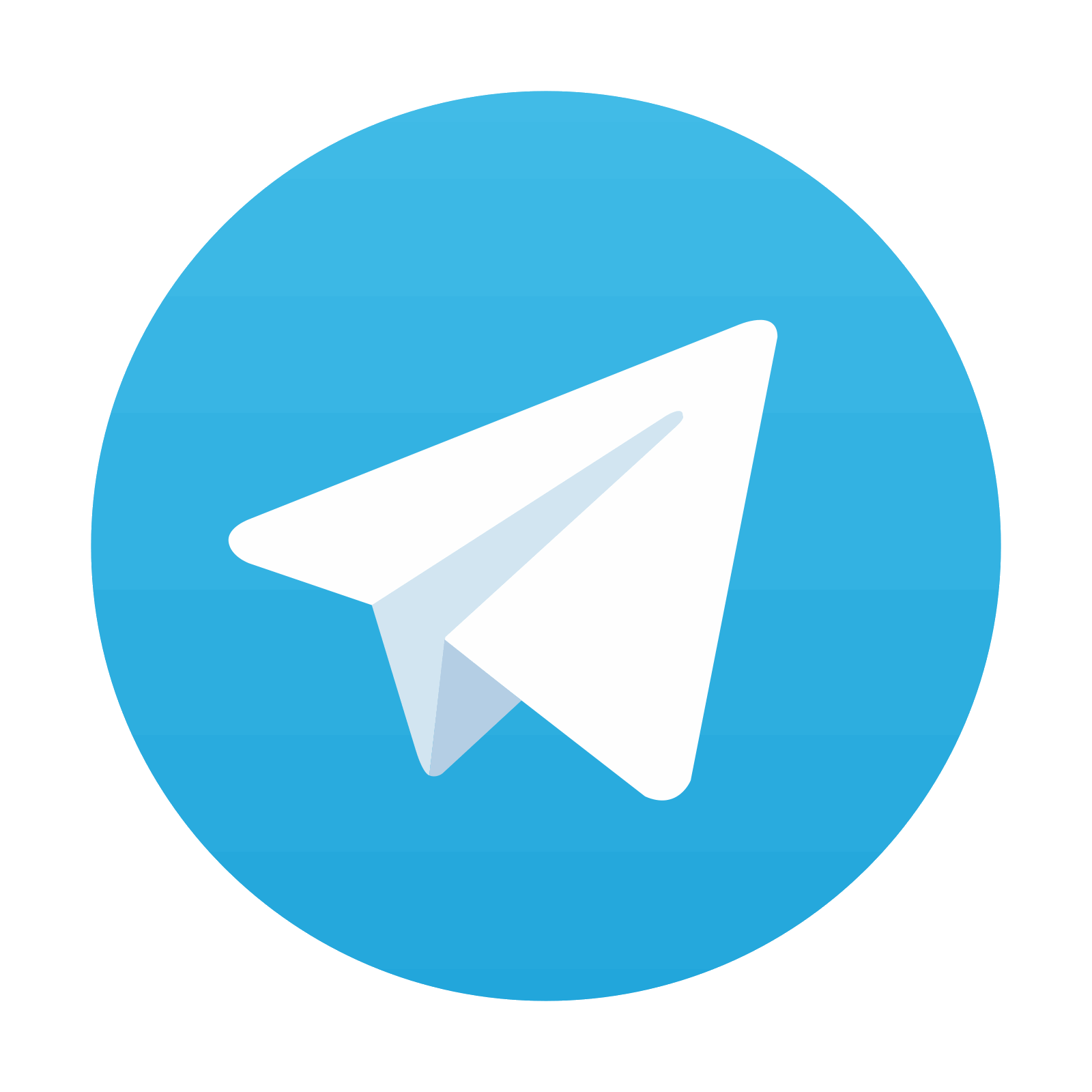
Stay updated, free articles. Join our Telegram channel
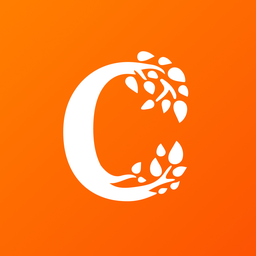
Full access? Get Clinical Tree
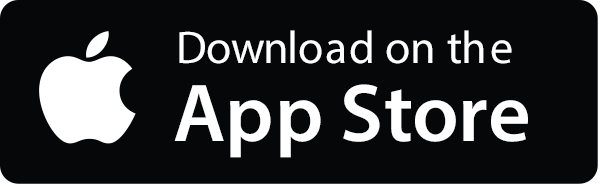
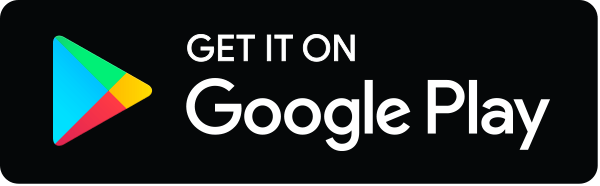