© Springer Science+Business Media New York 2016
Marina Kurian, Bruce M. Wolfe and Sayeed Ikramuddin (eds.)Metabolic Syndrome and Diabetes10.1007/978-1-4939-3220-7_66. Bile Acids, the Microbiome and Metabolic Disease-Implications for Surgery
(1)
Department of Surgery, University of Minnesota, 420 Delaware St. SE, MMC 195, Minneapolis, MN 55455, USA
Keywords
ObesityDiabetes mellitusHuman gut floraMetabolic pathwaysBacteroidetesPrevotellaRuminococcusBacterial richnessBacterial diversityMicrobiome diversity6.1 Obesity, Diabetes Mellitus, and the Microbiome
Perhaps one of the most exciting frontiers of medicine is the exploration of the human gut flora, or the microbiome. While it remains with potential as a target for future therapeutics, defining a healthy microbiome and pathologic variant remains a daunting task. In a pioneering study to further characterize healthy variation, the Human Microbiome Project Consortium in 2012 presented the microbiota of 242 healthy Western subjects from 18 different anatomic sites, including the distal gut, and while significant interindividual variation existed, metabolic pathways remained stable [1, 2]. As indicated by Arumugam et al., Bacteroidetes phyla dominate the gut microbiome. In their analysis, 33 samples across several nations formed three distinct clusters: Bacteroidetes, Prevotella, and Ruminococcus [3]. Generally, the gut microbiome is shared among family members with variation present in each individual’s microbial community [4]. Diet does have a critical impact [4–6]. As an example, in a comparison of microbiome in children from Europe and from rural Africa, the microbiome of African children displayed significantly increased bacterial richness and diversity, leading the authors to conclude that gut microbiome coevolved with their polysaccharide-rich diet [6].
6.2 Changes in the Microbiome in Obesity and T2DM
Obesity is associated with a significant decrease in microbiome diversity and richness [5]. In a study of obese and non-obese Danish individuals, obese individuals shared an “inflammatory” phenotype, having a higher prevalence of Bacteroides and R. gnavus, which have been associated with Inflammatory Bowel Disease [6–8]. Patients characterized as having a low gene count had a greater prevalence of obesity, insulin resistance, fatty liver, and low-grade inflammation than patients with a high gene count. Low gene count patients were also more prone to gaining more weight over time [5, 9]. In another study by Vrieze et al., male humans with metabolic syndrome were the recipients of either autologous microbiota or that of lean donors. Six weeks after small intestinal infusion, recipients from lean donors experienced increased insulin sensitivity, along with increased levels of butyrate-producing microbiota [10]. Perhaps one of the most telling studies was performed by Ridaura et al. who transplanted fecal microbiota from adult twin pairs discordant for obesity into germ-free mice. Not only were these obesity-associated phenotypes transmissible, cohousing with lean co-twin’s microbial recipient prevented increasing body mass and adverse metabolic outcomes [11]. Diet had an integral role, and when mice were fed a low-fat, high-fiber diet, they were protected against the effects of obese microbiota [12].
Correlations between shifts in the microbiome and obesity have been observed, although causality remains to be discerned. In general, one of the noteworthy observations has been the increased ratio of the phylum Firmicutes to the phylum Bacteroidetes seen with obesity [13–15]. This concept was initially proposed by Turnbaugh et al. who also demonstrated that obese microbiome has perhaps an increased capacity to harvest energy from the diet [16]. Further support for the Firmicutes to Bacteroidetes ratio was lent after the observation that this ratio is decreased with percentage reduction in body weight [13]. However, this observation has been met with some controversy, as other studies have failed to identify the difference in this ratio between obese and lean patients [17, 18]. Perhaps controversy in this regard lies in the fact that these are entire phylum changes, and more specific family and species levels changes should be pursed to characterize pathogen with phenotypic changes. For example, Zhang et al. assessed contributions of host genetics and diet in altering the microbiome in mice. Sixty-five species-level phylotypes were correlated with differences induced by diet, with diet explaining 57 % of the total structural variation in gut microbiome. Genetic mutation accounted for 12 %. Barrier-protecting Bifidobacterium species were nearly absent in all animals, with an observed increase in Desulfovibrionaceae, a sulfate-reducing and endotoxin-producing bacteria, in all animals with impaired glucose tolerance [19].
Not surprisingly, T2DM in humans is associated with microbial shifts as well. In a study by Qin et al., a protocol for a metagenome-wide association study was developed to study 345 Chinese individuals. Patients with T2DM portrayed moderate intestinal dysbiosis as indicated by a decrease in butyrate-producing Roseburia intestinalis and F. prausnitzii, along with an increase in opportunistic pathogens including Bacteroides caccae, Clostridiales, and Escherichia coli. Lean patients in this study exhibited an enriched population of butyrate-producing bacteria [20]. Similarly, Karlsson et al. studied the gut meganome in 145 European women with normal, impaired, or diabetic glucose control. This group similarly displayed increases in Clostridiales species, and decreases in Roseburia intestinalis and F. prausnitzii [21, 22]. They also illustrated increased Proteobacteria, increased expression of microbial genes involved in oxidative stress, and decreased genes involved in vitamin synthesis [9, 21, 22]. Another protective bacterium identified is Akkermansia municiphila, a mucin-degrading bacterium, negatively associated with type 1 and type 2 diabetes mellitus [20], while Bacteroidetes and Prevotella are increased in proportion to a decrease in Firmicutes and Clostridia [21–23].
6.3 Implicated Metabolic Pathways: LPS and Increased Gut Barrier Leak
Circulating lipopolysaccharide (LPS) is an integral and early step in the development of insulin resistance and diabetes having been observed in mice and humans [24–26]. Mice fed a high fat diet had two to three times increased levels of circulating LPS [26]. CD-14 mutant mice, which are resistant to LPS, are also resistant to high-fat diet-induced insulin resistance [26, 27]. Cani et al. administered antibiotics to mice on a high-fat diet, demonstrating that changes in the gut microbiome are responsible for endotoxemia and the subsequent inflammatory cascade, correlating strongly with intestinal permeability [27]. In patients with diabetes mellitus, LPS levels are significantly elevated, leading to increased secretion of pro-inflammatory cytokines [4, 28]. In mice fed a high-fat diet for 3 months, LPS was elevated in the presence of diabetes, and related this to increased gut permeability in the ileum and cecum [29]. Obese patients and rodents exhibit increased richness of LPS-producing bacteria, notably increased Enterobacteriacaea and Desulfovibrionaceae, corresponding with increased circulating LPS [30]. In obese human adolescents undergoing an exercise and diet program, fecal Enterobacteriaceae was significantly decreased [31, 32]. Once systemic, LPS binds LPS-binding protein and is recognized by CD14 and TLR4 [33, 34]. This in turn leads to a pro-inflammatory response and production of inflammatory cytokines potentially linking the microbiome to the diabetic phenotype [35].
6.4 Changes Following Bariatric Surgery
Following the gastric bypass, there is an increase in richness and diversity of the gut microbiome associated with white adipose tissue genes [36]. In a study comparing nine individuals, with three in each of normal weight, morbidly obese, and post-gastric bypass groups, Firmicutes was dominant in the first two groups, but decreased in post-gastric bypass individuals with a proportional increase in Gammaproteobacteria [15]. Akkermansia was also increased in the post-gastric bypass population. Graessler et al. compared patients before and 3 months following gastric bypass, having also observed a reduction in Firmicutes, with an increase in Proteobacteria. However, they also observed a decrease in F. prausnitzii correlating directly with fasting blood glucose levels [37]. Furet et al. observed an increase in F. Prausnitzii correlating with improved circulating CRP and IL6 in 30 patients with T2DM following gastric bypass. In an interesting and telling study performed by Liou et al., shifts observed following RYGB are conserved between humans, mice and rats, observing an increase in Gammaproteobacteria (Escherichia) and Verrucomicrobia (Akkermansia). Transferring gut microbiota from mice having undergone RYGB to germ-free mice resulted in weight loss and decreased fat mass relative to recipients of microbiota from mice following sham surgery [38].
In a study by Monte et al., 15 morbidly obese patients with T2DM underwent RYGB, with blood samples collected on day of surgery and 180 days after surgery. Interestingly, systemic inflammation as measured by multiple cytokines, including MCP-1 and CRP, and bacterial LPS were significantly reduced following surgery, possibly linking this improvement in systemic inflammation as a mechanism underlying metabolic improvement [39].
Certainly, further characterization of the microbiome is required; more important may be the role species have in altering gut physiology translating to systemic changes, particularly in diabetes. Much of this may involve gaining a stronger understanding of gut immunology at the enterocyte level given the known systemic inflammation that characterizes white adipose tissue in T2DM.
6.5 Possible Mediators of Change
6.5.1 Bile Acids
Obese patients exhibit an attenuated fasting and post-prandial bile acid response compared to lean individuals [40]. Following RYGB, both fasting and post-prandial plasma bile acid responses are increased, and have been shown to correlate with improvement in glucose metabolism [41, 42]. Bile acids serve as ligands for both G-protein-coupled receptor TGR5, which increases GLP-1 production, and nuclear hormone receptor farnesoid X receptor (FXR) [43]. FXR induces formation of fibroblast growth factor-19, which enters portal circulation and inhibits bile acid synthesis via CYP7A1. Primary bile acids cholic acid and chenodeoxycholic acids are synthesized from cholesterol in the liver via CYP7A1. In the gut, the microbiome converts primary bile acids into secondary bile acids by dehydroxylation, creating deoxycholic and lithocholic acids. Greater than 95 % of bile acids are reabsorbed by the gut and are transported back to the liver [40, 44, 45].
In an interesting study performed at the University of Cincinnati, mouse FXR knockout models undergoing vertical sleeve gastrectomy (VSG) highlighted the significance of the FXR receptor and the bile acid pathway in mediating metabolic improvement. FXR knockouts portray substantially reduced weight loss and decreased improvement in glucose tolerance following VSG. Along with these observations, changes in microbiome were also observed between wild-type mice undergoing VSG, and FXR knockouts undergoing VSG. Knockouts showed decreased abundance of one genus in the Porphyromonadaceae family in Knockout-VSG model. Roseburia was also increased in wild-type VSG model relative to wild-type shams, while no difference was observed between the knockout and sham FXR knockout models [46].
The intimate and complex relationship between bile acids and the microbiome is one that requires further research as we continue toward identifying physiologic roles for the multitude of bacterial species that comprise our gut microbiome.
6.5.2 Short-Chain Fatty Acids
Short-chain fatty acids (SCFAs) including acetate, butyrate, and propionate are main colonic bacterial fermentation products that serve as main energy sources and have been established as essential nutrients acting as signaling molecules [47, 48]. Two orphan G-protein-coupled receptors, FFAR2 (formerly gpr 3) and FFAR3 (formerly gpr41), have been identified as being activated by SCFAs [49].
At this time, it is appreciated that the SCFA–FFAR2 interaction has a significant effect on inflammatory responses. Priopionate and butyrate have been observed to reduce low-grade inflammation and increase leptin [50, 51]. Butyrate-producing bacteria have been particularly implicated in studies involving fecal transplantation as a therapeutic modality for insulin resistance [52]. Oral administration of acetate and propionate reduced glycemia in diabetic KK-A (y) and wild-type rats [53]. Interestingly, incubation of human colonic epithelial cell line with butyrate has shown to increase transepithelial resistance by promoting assembly of tight junction, highlighting a potential correlation with metabolic endotoxemia and gut barrier leak highlighted earlier [54]. Along these lines, propionate and butyrate reduce expression of pro-inflammatory cytokines TNF-α and IL-6 in human adipose tissue, while butyrate has been shown to increase the secretion of the anti-inflammatory cytokine IL-10 by human monocytes exposed to bacteria [55, 56].
Substantial knowledge has been gained from mouse knockout models as well. FFAR2 knockout mice display exacerbated or unresolved inflammation in models of colitis, arthritis, and asthma [57]. Kimura et al. demonstrated that FFAR2 knockout mice are obese on a normal diet, whereas mice overexpressing FFAR2 remain lean even on a high fat diet. This difference is abolished under germ-free conditions or after antibiotics. Furthermore, SCFA-mediated activation of FFAR2 suppresses insulin signaling in adipocytes thus inhibiting fat accumulation in adipose tissue [58]. De Vadder et al. were able to show that perhaps one mechanism by which SCFAs, particularly propionate and butyrate, mediate their influence is via activation of intestinal gluconeogenesis (IGN). Butyrate activates IGN gene expression via a cAMP-dependent mechanism, while propionate interestingly activates IGN via a gut-brain neural circuit involving FFAR3. This relationship is abolished with capsaicin-induced periportal nervous deafferentiation in rats [59].
Altogether, as more data are accumulating, it is becoming clearer the impact the gut microbiome has on human metabolism, particularly through SCFAs. How we may utilize these findings from a therapeutic perspective in alleviating diabetes remains to be seen.
6.6 Conclusion
Interest in the microbiome has increased considerably over the past few years, especially with the discovery of a divergence and pattern observed in obese and diabetic humans relative to lean and healthy humans. These changes in the microbiome strongly correlate along the spectrum of metabolic disease. How the human gut interacts with specific bacterial species remains a significant, but necessary challenge to overcome. We, in the field of bariatric surgery, have an important role in elucidating this puzzle given our significant capacity to alter the microbiome following surgery. These changes clearly correlate with the dramatic metabolic improvement following bariatric surgery. The challenge has always been to isolate important physiologic changes following surgery, and relating them to the well-established phenotypic changes that occur. This is no different as it relates to the human gut microbiome.
References
1.
Human Microbiome Project Consortium. Structure, function and diversity of the healthy human microbiome. Nature. 2012;486(7402):207–14. doi:10.1038/nature11234.CrossRef
2.
Sweeney TE, Morton JM. The human gut microbiome: a review of the effect of obesity and surgically induced weight loss. JAMA Surg. 2013;148(6):563–9. doi:10.1001/jamasurg.2013.5.PubMedCentralCrossRefPubMed
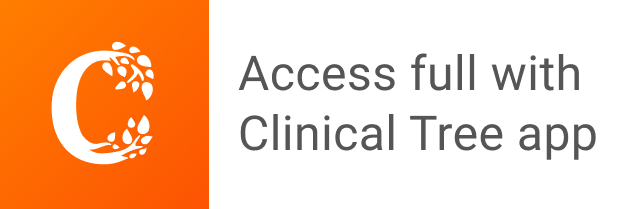