Thermal ablation, which induces irreversible cellular injury from focal high-temperature tissue heating that is generated from a focal energy source, has become an accepted treatment option for focal primary and secondary malignancies in a wide range of organs including the liver, lung, kidney, bone, and adrenal glands. Given the rising complexity of treatment types and paradigms in oncology, and the wider application of thermal ablation techniques and adjuvant therapy reviewed in this article, a thorough understanding of the basic principles and recent advances in thermal ablation is a necessary prerequisite for their effective clinical use.
Thermal ablation has become an accepted treatment option for focal primary and secondary malignancies in a wide range of organs including the liver, lung, kidney, bone, and adrenal glands. The largest clinical experience has been for hepatic malignancies, where long-term outcomes similar to surgical resection have been reported in some matched patient populations. Benefits of minimally invasive, image-guided ablative techniques include reduced cost and morbidity compared with standard surgical resection, and the ability to treat patients who are not surgical candidates. However, limitations in thermal ablative efficacy exist, including persistent growth of residual tumor at the ablation margin, the inability to effectively treat larger tumors, and variability in complete treatment based on tumor location. Extensive investigation into potential strategies to improve ablation outcomes continues, and focuses on technological development of ablative systems, improving ablative predictability, and combining thermal ablation with additional therapies such as chemotherapy and radiation. Given the rising complexity of treatment types and paradigms in oncology, and the wider application of thermal ablation techniques, a thorough understanding of the basic principles and recent advances in thermal ablation is a necessary prerequisite for their effective clinical use.
Goals of minimally invasive thermal tumor ablation
The overall goal of minimally invasive thermal tumor ablation for focal malignancies encompasses several specific objectives, regardless of the specific thermal ablative device that is used. The primary purpose of treatment is to completely eradicate all viable malignant cells within the target tumor. Based on tumor recurrence patterns in long-term studies in patients who have undergone surgical resection, and more recently, ablation, along with studies that have performed pathologic analysis of resection margins, there is often viable persistent microscopic tumor foci in a rim of apparently normal surrounding parenchymal tissue beyond the visible tumor margin. Therefore, tumor ablation therapies also attempt to include a 5- to 10-mm “ablative” margin of normal surrounding tissue in the target zone, though the required thickness of this margin is variable based on tumor and organ type. While complete treatment of the target tumor is of primary importance, specificity and accuracy is also highly preferred, with a secondary goal of incurring as little injury as possible to surrounding nontarget normal tissue. This ability to minimize damage to normal organ parenchyma is one of the significant advantages of minimally invasive percutaneous thermal ablation, and can be critical in patients who have focal tumors in the setting of limited functional organ reserve. Examples of clinical situations where this is relevant include focal hepatic tumors in patients with underlying cirrhosis and limited hepatic reserve, patients with Von Hippel Lindau syndrome who have limited renal function and require treatment of multiple renal tumors, and patients with primary lung tumors with extensive underlying emphysema and limited lung function. Many of these patients are not surgical candidates because of limited native organ functional reserve placing them at a higher risk for postoperative complications or organ failure. An additional consideration is that appropriate and complete tumor destruction occurs only when the entire target tumor is exposed to appropriate temperatures, and is therefore determined by the pattern of tissue heating in the target tumor. For larger tumors (usually defined to be larger than 3–5 cm in diameter), a single ablation treatment may not be sufficient to entirely encompass the target volume. In these cases, multiple overlapping ablations or simultaneous use of multiple applicators may be required to successfully treat the entire tumor and achieve an ablative margin, although accurate targeting and probe placement can often be technically challenging ( Fig. 1 ). Finally, growth patterns of the tumor itself can influence overall treatment outcomes, with slow-growing tumors more amenable to multiple treatment sessions over longer periods of time. These principles are applicable to a wide range of ablative technologies, including both thermal and nonthermal strategies.
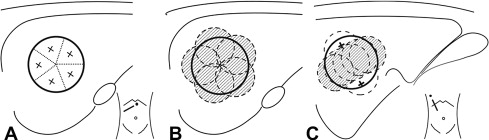
Principles of tissue heating in thermal ablation
Thermal ablation induces irreversible cellular injury from focal high-temperature tissue heating that is generated from a focal energy source. Multiple energy sources have been used to provide the heat necessary to induce coagulation necrosis. Focal high temperature (generally accepted as >50°C) ablation therapies use radiofrequency (RF), microwave, laser, or ultrasound energies to generate isolated increases in tissue temperature. Historically, the majority of clinical and experimental studies have been performed using RF-based ablative devices. As such, this review of basic principles of focal high temperature ablative therapies will primarily use RF systems as a representative model in describing the basic principles of focal thermal ablation, with discussions of other energy-based technologies where relevant.
Tissue heating occurs though 2 specific mechanisms. First, an applicator placed within the center of the target tumor delivers energy that interacts with tissue to generate focal heat immediately around it. This approach is similar for all thermal ablation strategies, regardless of the type of energy source used, though specific mechanisms of heat induction are energy specific. For example, as RF current travels from the electrode applicator to the remote grounding pad, local tissue resistance to current flow results in ionic agitation and heat generation. In microwave-based systems, the needle antenna applies electromagnetic energy to the tissue, and as molecules with an intrinsic dipole moment (such as water) are forced to continuously align to the externally applied magnetic field, the kinetic energy that is generated results in local tissue heating. Laser ablation uses emission of laser energy from optic fibers to generate tissue heat immediately around the fiber tip. Ultrasound-based systems induce tissue heating by applying a focused beam of ultrasound energy with a high peak intensity, either directly around a percutaneously placed applicator (like for other ablative systems), or transcutaneously by directing several ultrasound beams of lower intensity from different directions so that they converge at the target tumor, where the ultrasound energy is absorbed by the tissue and converted to heat. The second mechanism of tissue heating in thermal ablation uses thermal tissue conduction. Heat generated around the electrode diffuses through the tumor and results in additional high-temperature heating that is separate from the direct energy-tissue interactions that occur around the electrode. The contribution of thermal conduction to overall tissue ablation is determined by several factors. Tissue heating patterns vary based on the specific energy source used—for example, microwave-based systems induce tissue heating at a much faster rate than RF-based systems, and so thermal conduction contributes less to overall tissue heating. In addition, tumor and tissue characteristics also affect thermal conduction. As an example, primary hepatic tumors (hepatocellular carcinoma) transmit heat better than the surrounding cirrhotic hepatic parenchyma.
Regardless of the energy source used, the end point of thermal ablation is adequate tissue heating so as to induce coagulative necrosis throughout the defined target area. Relatively mild increases in tissue temperature above baseline (40°C) can be tolerated by normal cellular homeostatic mechanisms. Low-temperature hyperthermia (42°C–45°C) results in reversible cellular injury, though this can increase cellular susceptibility to additional adjuvant therapies such as chemotherapy and radiation. Irreversible cellular injury occurs when cells are heated to 46°C for 60 minutes, and occurs more rapidly as the temperature rises, so that most cell types die in a few minutes when heated at 50°C. Immediate cellular damage centers on protein coagulation of cytosolic and mitochondrial enzymes and nucleic acid-histone protein complexes, which triggers cellular death over the course of several days. “Heat fixation” or “coagulation necrosis” is used to describe this thermal damage, even though ultimate manifestations of cell death may not fulfill strict histopathologic criteria of coagulative necrosis. This has implications with regard to clinical practice, as percutaneous biopsy and standard histopathologic interpretation may not be a reliable measure of adequate ablation. Therefore, optimal temperatures for ablation range likely exceed 50°C. At the other end of the temperature spectrum, tissue vaporization occurs at temperatures higher than 110°C, which in turn limits further current deposition in RF-based systems (as compared with, for example, microwave systems that do not have this limitation).
The exact temperature at which cell death occurs is multifactorial and tissue specific. Based on prior studies demonstrating that tissue coagulation can be induced by focal tissue heating to 50°C for 4 to 6 minutes, this has become the standard surrogate end point for thermal ablation therapies in both experimental studies and current clinical paradigms. However, studies have shown that depending on heating time, the rate of heat increase, and the tissue being heated, maximum temperatures at the edge of ablation are variable. For example, maximum temperatures at the edge of ablation zone, known as the “critical temperature,” have been shown to range from 30°C to 77°C for normal tissues and from 41°C to 64°C for tumor models (a 23°C difference). Likewise, the total amount of heat administered for a given time, known as the thermal dose, varies significantly between different tissues. Thus, the threshold target temperature of 50°C should be used only as a general guideline.
Applying the principles of the Bioheat equation to achieve meaningful volumes of tumor ablation
Early studies using RF-based systems demonstrated that percutaneous thermal ablation with a single-electrode system was inadequate for tumors larger than 1.8 cm. Subsequent investigation (such as the techniques described later) has increased the size of consistently achievable ablation to at least 3 to 5 cm in diameter. However, with the continued expansion of the use of thermal ablation techniques into new tumor types, and the growing need for effective alternative treatment options in patients with larger tumors and who are ineligible for surgery, the need to reliably ablate larger tumors persists.
Complete and adequate destruction by thermal ablation requires that the entire tumor (and usually an ablative margin) be subjected to cytotoxic temperatures. Success of thermal ablation is contingent on adequate heat delivery. The ability to heat large volumes of tissue in different environments is dependent on several factors encompassing both energy delivery and local physiologic tissue characteristics. The relationship between this set of parameters, as described by the Bioheat equation, can be simplified to describe the basic relationship guiding thermal ablation–induced coagulation necrosis as: “coagulation necrosis = (energy deposited × local tissue interactions) − heat loss.” Based on this relationship, several strategies to increase the ability to ablate larger tumors have been concurrently pursued. These encompass (1) technological developments, including modification of energy input algorithms and electrode design to deposit more energy into the tissue, (2) improved understanding and subsequent modification of the biophysiologic environment to increase tissue heating, and (3) incorporation of adjuvant therapies to increase uniformity of tumor cellular injury in the ablation zone, along with increasing cellular destruction in the nonlethal hyperthermic zone around the ablation.
Technologic advancements
Most investigation to improve thermal ablation outcomes has focused on device development, much of which has been, as noted, for RF-based ablation systems. Technologic efforts to increase ablation size have focused on modifying energy deposition algorithms and electrode designs to increase both the amount of tissue exposed to the active electrode and the overall amount of energy that can be safely deposited into the target tissue.
Refinement of Energy Application Algorithms
The algorithm by which energy is applied during thermal ablation is dependent on the energy source, device, and type of electrode that is being used. While initial power algorithms for RF-based systems were based on a continuous and constant high-energy input, tissue overheating and vaporization ultimately interferes with continued energy input because of high impedance to current flow from gas formation. Therefore, several strategies to maximize energy deposition have been developed and, in some cases, incorporated into commercially available devices.
Applying high levels of energy in a pulsed manner, separated by periods of lower energy, is one such strategy that has been used with RF-based systems to increase the mean intensity of energy deposition. If a proper balance between high and low energy deposition is achieved, preferential tissue cooling occurs adjacent to the electrode during periods of minimal energy deposition without significantly decreasing heating deeper in the tissue. Thus, even greater energy can be applied during periods of high energy deposition, thereby enabling deeper heat penetration and greater tissue coagulation. Synergy between a combination of internal cooling of the electrode and pulsing has resulted in even greater coagulation necrosis and tumor destruction than either method alone. Pulsed-energy techniques have also been successfully used for microwave and laser-based systems.
Another strategy is to slowly increase (or “ramp-up”) the RF energy application in a continuous manner, until the impedance to RF current flow increases prohibitively. This approach is often paired with multitined expandable electrodes, which have a greater contact surface area with tissue, and in which the goal is to achieve smaller ablation zones around multiple small electrode tines. This algorithm is also often combined with a staged expansion of the electrode system such that each small ablation occurs in a slightly different location within the tumor (with the overall goal being ablating the entire target region).
“Electrode switching” is an additional technique that is incorporated into pulsing algorithms to further increase RF tissue heating. In this, multiple independently placed RF electrodes are connected to a single RF generator, and RF current is applied to a single electrode until an impedance spike is detected, at which point current application is applied to the next electrode, and so forth. Several studies have demonstrated significant increases in ablation zone size and a reduction in application time using this technique. For example, Brace and colleagues also show larger and more circular ablation zones with the switching application, with more rapid heating being 74% faster than the sequential heating (12 vs 46 minutes).
Finally, continued device development has also led to increases in the overall maximum amount of power that can be delivered. For RF-based devices, the maximum amount of RF current that can be delivered is dependent on both the generator output and the electrode surface area, as higher surface areas reduce the current density and therefore, adequate tissue heating around the electrode cannot be achieved. Whereas initial systems had maximum power outputs of less than 200 W, subsequent investigation suggests that higher current output and larger ablation zones can be achieved if higher-powered generators are coupled with larger-surface area electrodes. For example, Solazzo and colleagues used a 500-KHz (1000 W) high-powered generator in an in vivo porcine model and achieved larger coagulation zones with a 4-cm tip cluster electrode (5.2 ± 0.8 cm) compared with a 2.5-cm cluster electrode (3.9 ± 0.3 cm). Similar gains in ablation size have been seen in higher-powered versions of microwave-based systems.
Electrode Modification
Development of ablation applicators (electrodes for RF, antennae for microwave, and diffuser tips for laser-based systems) has contributed significantly to the ability to reliably achieve larger ablation zones. Several strategies to increase the amount of energy deposition and the overall ablation size have been balanced with the need for smaller-caliber electrodes to permit the continued use of these devices in a percutaneous and minimally invasive manner. These include the use of multiple electrodes simultaneously, either adjacent to each other or as part of an expandable device through a single introducer needle, the use of cooling systems to minimize tissue and electrode overheating, and the use of bipolar systems for RF ablation to increase tissue heating in the target zone.
Originally, simply lengthening the electrode tip increased coagulation in an asymmetric and preferentially longitudinal geometry. Use of a single electrode inserted multiple times to perform overlapping ablations requires significantly greater time and effort, making it impractical for routine use in a clinical setting. Therefore, the use of multiple electrodes simultaneously either in a pre-set configuration represents a significant step forward in increasing overall ablation size. Initial work with multiple electrodes demonstrated that placement of several monopolar electrodes in a clustered arrangement (no more than 1.5 cm apart) with simultaneous RF application could increase coagulation volume by more than 800% compared with a single electrode. Subsequently, working to overcome the technical challenges of multiprobe application, multitined expandable RF electrodes have been developed. These systems involve the deployment of a varying number of multiple thin, curved tines in the shape of an umbrella or more complex geometries from a central cannula. This surmounts earlier difficulties by allowing easy placement of multiple probes to create large, reproducible volumes of necrosis. Leveen, using a 12-hook array, was able to produce lesions measuring up to 3.5 cm in diameter in in vivo porcine liver by administering increasing amounts of RF energy from a 50-W RF generator for 10 minutes. More recently, Applebaum and colleagues were able to achieve greater than 5 cm of coagulation in in vivo porcine liver using currently commercially available expandable electrodes with optimized stepped-extension and -power input algorithms.
Although most conventional RF systems use monopolar electrodes (where the current runs to remotely placed grounding pads placed on the patient’s thighs to complete the circuit), several studies have reported results using bipolar arrays to increase the volume of coagulation created by RF application. In these systems, applied RF current runs from an active electrode to a second grounding electrode in place of a grounding pad. Heat is generated around both electrodes, creating elliptical lesions. McGahan and colleagues used this method in ex vivo liver to induce necrosis of up to 4.0 cm in the long-axis diameter, but could only achieve 1.4 cm of necrosis in the short-axis diameter. Although this increases the overall size of coagulation volume, the shape of necrosis is unsuitable for actual tumors, making the gains in coagulation less clinically significant. Desinger and colleagues have described another bipolar array that contains both the active and return electrodes on the same 2-mm diameter probe. Lee and colleagues used 2 multitined electrodes as active and return electrodes to increase coagulation during bipolar RF ablation. Finally, several studies have used a multipolar (more than 2) array of electrodes (multitined and single internally cooled) during RF ablation to achieve even greater volumes of coagulation.
One of the limitations for RF-based systems has been overheating surrounding the active electrode, leading to tissue charring, rising impedance, and RF circuit interruption, ultimately limiting overall RF energy deposition. One successful strategy to address this has been the use of internal electrode cooling, whereby electrodes contain 2 hollow lumens that permit continuous internal cooling of the tip with a chilled perfusate, and the removal of warmed effluent to a collection unit outside of the body. This reduces heating directly around the electrode, tissue charring, and rising impedance, allows greater RF energy deposition, and shifts the peak tissue temperature farther into the tumor, contributing to a broader depth of tissue heating from thermal conduction. In initial studies using cooling of 18-gauge single or clustered electrode needles with chilled 0°C saline perfusate, significant increases in RF energy deposition and ablation zone size were observed compared with conventional monopolar uncooled electrodes in ex vivo liver, with findings subsequently confirmed in in vivo large animal models and clinical studies. Similar results were observed when chilled saline was infused in combination with expandable electrode systems (referred to as an “internally cooled wet electrode”), though infusion of fluid around the electrode is more difficult to control and makes the reproducibility of results more variable. Most recently, several investigators have used alternative cooling agents (for example, argon or nitrogen gas) to achieve even greater cooling, and therefore larger zones of ablation, around the RF electrode tip. As for RF-based systems, cooling of antennae shafts for microwave applicators has also been developed, which reduces shaft heating (and associated complications such as skin burns) while allowing increased power deposition through smaller-caliber antennae.
Although many of these technological advances have been developed independently of each other, they may often be used concurrently to achieve even larger ablation zones. Furthermore, while much of this work has been based on RF technology, specific techniques can be used for other thermal ablative therapies. For example, multiple electrodes and applicator cooling have been effectively applied in microwave-based systems as well.
Modification of the biophysiologic environment
Ablation research has traditionally focused on creating larger or more uniform reproducible zones of ablation through device and application development. However, device development is ultimately constrained by tumor and organ physiology. As such, many recent investigations have centered on altering underlying tumor physiology as a means to advance thermal ablation. Most studies to date have focused on the effects of tissue characteristics in the setting of temperature-based therapies in general (such as tissue perfusion and thermal conductivity), and system-specific characteristics, such as electrical conductivity for RF-based ablation.
Tissue Perfusion
The foremost factor limiting thermal ablation of tumors continues to be tissue blood flow, for which the effects are twofold. First, larger-diameter blood vessels with higher flow act as heat sinks, drawing away either heat (or cold) from the ablative area ( Fig. 2 ). For example, in a study in an in vivo porcine model, Monsky and colleagues examined the effect of hepatic vessel diameter on RF ablation outcome. Using computed tomography (CT) and histopathologic analysis, more complete thermal heating and a reduced heat-sink effect was identified when hepatic vessels within the heating zone were less than 3 mm in diameter. In contrast, vessels greater than 3 mm in diameter had higher patency rates, less endothelial injury, and greater viability of surrounding hepatocytes after RF ablation. This strong predictive nature of hepatic blood flow on the extent of RF induced coagulation has been confirmed in multiple studies where increased coagulation volumes have been obtained when hepatic blood flow is decreased, either by balloon or coil embolization or the Pringle maneuver. The second effect of tissue vasculature is a result of perfusion-mediated tissue cooling (capillary vascular flow) that also functions as a heat sink. By drawing heat from the treatment zone, this effect reduces the volume of tissue that receives the required minimal thermal dose for coagulation. Along with the use of mechanical occlusion, several studies have also used pharmacologic alteration of tissue perfusion to reduce these effects. Goldberg and colleagues modulated hepatic blood flow using intra-arterial vasopressin and high-dose halothane in conjunction with RF ablation in in vivo porcine liver. Arsenic trioxide, which has recently received increasing attention as a novel antineoplastic agent, has been shown to preferentially decrease tumor blood flow and significantly increase RF-induced coagulation in a renal tumor model. More recently, promising antiangiogenic therapies, such as sorafenib, are also starting to be studied as combination therapies with ablation, with similar encouraging effects in animals leading to the initiation of clinical trials.
Thermal Conductivity
Initial clinical studies using RF ablation for hepatocellular carcinoma in the setting of underlying cirrhosis noted an “oven” effect (ie, increased heating efficacy for tumors surrounded by cirrhotic liver or fat, such as exophytic renal cell carcinomas), or altered thermal transmission at the junction of tumor tissue and surrounding tissue. Subsequent experimental studies in ex vivo agar phantoms and bovine liver have confirmed the effects of varying tumor and surrounding tissue thermal conductivity on effective heat transmission during RF ablation, and have further demonstrated the role of “optimal” thermal conductivity characteristics on ablation outcomes. For example, very poor tumor thermal conductivity limits heat transmission centrifugally away from the electrode with marked heating in the central portion of the tumor, and limited, potentially incomplete heating in peripheral portions of the tumor. By contrast, increased thermal conductivity (such as in cystic lesions) results in fast heat transmission (ie, heat dissipation), with potentially incomplete and heterogeneous tumor heating. Furthermore, in recent agar phantom and computer modeling studies, Liu and colleagues demonstrated that differences in thermal conductivity between the tumor and surrounding background tissue (specifically, decreased thermal conductivity from increased fat content of surrounding tissue) results in increased temperatures at the tumor margin. However, heating was limited in the surrounding medium, making a 1-cm “ablative” margin difficult to achieve. An understanding of the role of thermal conductivity, and tissue- and tumor-specific characteristics, on tissue heating may be useful when trying to predict ablation outcome in varying clinical settings (eg, in exophytic renal cell carcinomas surrounded by perirenal fat, lung tumors surrounded by aerated normal parenchyma, or osseous metastases surrounded by cortical bone).
Electrical Conductivity
Local electrical conductivity is a tissue characteristic that specifically influences energy deposition in RF-based systems. RF-induced tissue heating, generated by resistive heating from ionic agitation, is strongly dependent on the local electrical conductivity. To this end, the effect of local electrical conductivity on RF-induced tissue heating can be broadly divided into 2 categories. First, altering the electrical environment immediately around the RF electrode with ionic agents can increase electrical conductivity before or during RF ablation. The increase in conductivity allows greater energy deposition and, therefore, increased coagulation volume. Saline may also be of benefit when attempting to ablate cavitary lesions that might not otherwise contain a sufficient current path. In general, small volumes of highly concentrated sodium ions are injected in and around the ablation site to maximize local heating effects, findings observed in both experimental and clinical studies and subsequently incorporated into electrode development. However, it should be noted that saline infusion is not always a predictable process, as fluid can migrate to unintended locations and cause complications if not used properly.
Second, differences in electrical conductivity between the tumor and surrounding background organ can affect tissue heating at the tumor margin. Several studies have demonstrated increases in tissue heating at the tumor-organ interface when the surrounding medium is characterized by reduced lower electrical conductivity. In certain clinical settings, such as treating focal tumors in either lung or bone, marked differences in electrical conductivity may result in variable heating at the tumor/organ interface, and indeed limit heating in the surrounding organ, and may make obtaining a 1.0-cm ablative margin difficult.
Finally, electrical conductivity must be taken into account when using techniques such as hydrodissection to protect adjacent organs. Nonionic fluids can be used to protect tissues adjacent to the ablation zone (such as diaphragm or bowel) from thermal injury. For this application, fluids with low ion content, such as 5% dextrose in water (D5W), should be used because they have been proven to electrically force RF current away from the protected organ, decrease the size and incidence of burns on the diaphragm and bowel, and reduce pain scores in patients treated with D5W when compared with ionic solutions, such as saline. Ionic solutions such as 0.9% saline should not be used for hydrodissection because, as noted, they actually increase RF current flow.
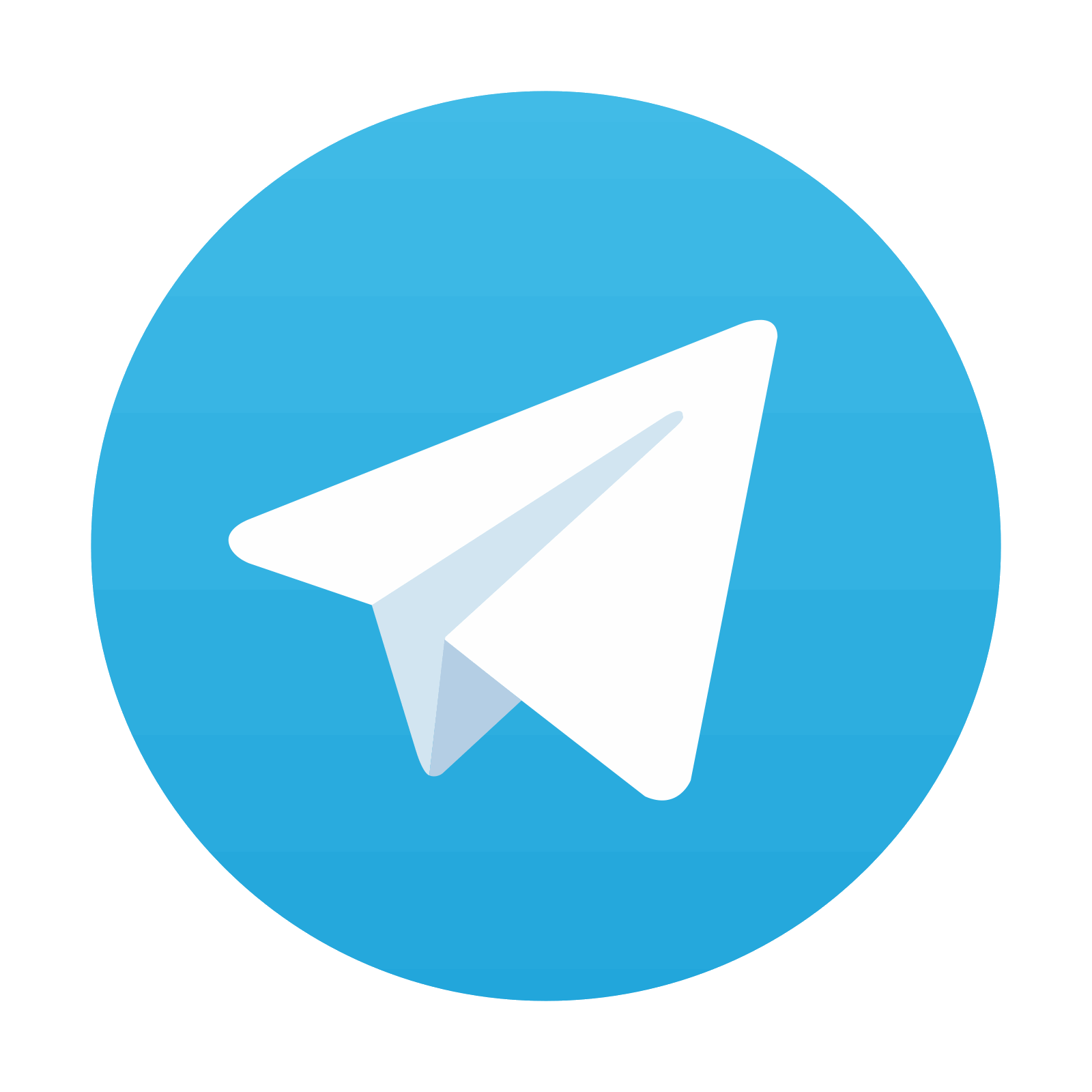
Stay updated, free articles. Join our Telegram channel
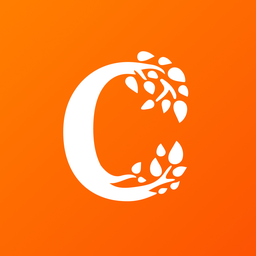
Full access? Get Clinical Tree
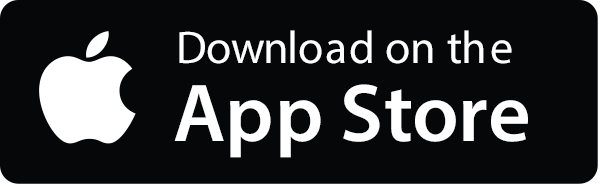
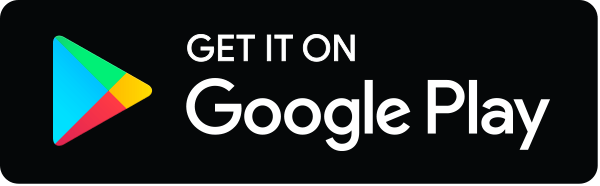