Key points
- 1.
Radiation therapy is used for the treatment of primary and metastatic gynecologic malignancies.
- 2.
Radiation therapy depends on the differential susceptibility of tumors to irradiation compared with normal tissues, and research attempts to maximize the therapeutic ratio are ongoing.
- 3.
Photons, a nonparticulate form of radiation, are the primary modality of therapeutic irradiation used in gynecologic carcinomas.
- 4.
Particulate irradiation with electrons and protons is also used in many centers but has specialized indications.
- 5.
Radiation is measured in international system (SI) unit of centigray (cGy) and gray (Gy). A typical dose for therapeutic irradiation for adjuvant therapy is 45 to 50 Gy and for gross disease, as in cervical carcinoma, is 80 to 85 Gy.
- 6.
Radiation is primarily generated from human-made sources, as in a linear accelerator, called x-rays, or from naturally radioactive decay, termed gamma rays. Therapeutically these are indistinguishable, although photons or electrons may be the therapeutic source.
- 7.
A variety of DNA lesions are produced by radiation including base damage, DNA–protein crosslinking, single-strand breaks, and double-strand breaks.
- 8.
There are two main forms of therapeutic radiation: External-beam radiation therapy delivers radiation to the body from an external source. Brachytherapy delivers radiation from sources that are placed within the body.
- 9.
An important concept, crucial to the delivery of brachytherapy, is that the dose of radiation at a given point is inversely proportional to the square of the distance from the source of radiation (dose ∝ 1/Distance 2 ). This allows the delivery of high therapeutic doses directly to the tumor and safe doses to adjacent critical organs. External methods of delivery cannot duplicate this and a high level of expertise is required for such treatments.
- 10.
Low-dose-rate brachytherapy has been replaced in most centers by high-dose-rate brachytherapy or pulse-dose-rate brachytherapy.
- 11.
Image guidance with computed tomography (CT), magnetic resonance imaging, or positron emission CT scans, in all aspects of radiation therapy (planning, external, and brachytherapy), increases the therapeutic ratio.
- 12.
Concurrent therapies (radiosensitizers and protectors) seek to increase this therapeutic ratio, each with its own mechanism of action to be exploited.
- 13.
The short- and long-term effects of irradiation are not trivial, and a risk-to-benefit discussion is crucial to the patient and multidisciplinary oncology team. During and after therapy, a team approach best serves the patient.
- 14.
Intensity-modulated radiation therapy, image-guided radiation therapy, and stereotactic radiation therapy are all tools in an increasingly complex toolbox for the radiation oncologist to use for increased local control, survival, and palliation. Ongoing studies evaluate the risk-to-benefit ratio and cost effectiveness of these new and expensive technologies.
A basic understanding of the principles of radiation oncology is essential to gynecologic oncologists. Radiation therapy is used in the curative treatment of locoregionally advanced cervical and vaginal carcinoma. It is often an adjuvant therapy prescribed for uterine or vulvar carcinoma and in inoperable patients may be the only definitive therapy available. Lastly, it can be used for the palliative treatment of gynecologic carcinomas.
Introduction to electromagnetic radiation
Radiant energy or radiation is an essential component of life on earth. For example, sunlight provides heat, light, and energy for plant photosynthesis, and radio waves provide a method of communication. This electromagnetic radiation physically consists of photons, or “packets” of energy without mass or charge. The primary difference between the radiations is the energy of the photons. The energy is proportional to frequency (E = hν, where h is Planck’s constant and ν is frequency) and inversely proportional to wavelength ( Table 22.1 ). A common analogy is to compare wavelength to the length of a person’s stride when walking; the number of strides per minute is the frequency of the wave.
Wavelength (M) | Frequency (Hz) | Energy (J) | |
---|---|---|---|
Radio | >1 × 10 −1 | <3 × 10 9 | <2 × 10 −24 |
Microwave | 1 × 10 −3 –1 × 10 −1 | 3 × 10 9 –3 × 10 11 | 2 × 10 −24 –2 × 10 −22 |
Infrared | 7 × 10 −7 –1 × 10 −3 | 3 × 10 11 –4 × 10 14 | 2 × 10 −22 –3 × 10 −19 |
Visible | 4 × 10 −7 –7 × 10 −7 | 4 × 10 14 –7.5 × 10 14 | 3 × 10 −19 –5 × 10 −19 |
Ultraviolet | 1 × 10 −8 –4 × 10 −7 | 7.5 × 10 14 –3 × 10 16 | 5 × 10 −19 –2 × 10 −17 |
X-ray | 1 × 10 −11 –1 × 10 −8 | 3 × 10 16 –3 × 10 19 | 2 × 10 −17 –2 × 10 −14 |
Gamma ray | <1 × 10 −11 | >3 × 10 19 | >2 × 10 −14 |
Photons, a nonparticulate radiation, are only one form of radiation. Another form of radiation is particulate radiation. Particulate radiation consists of subatomic particles such as electrons, protons, α particles, and neutrons.
Radiation is not harmful in ordinary quantities and is actually helpful to life processes. In fact, exposure to radiation is a constant phenomenon ( Tables 22.2 and 22.3 ). However, high-energy, or “ionizing,” radiation is not entirely harmless, although it is commonly used for both diagnostic and therapeutic purposes. High-energy radiation can be injurious to biologic material, and its use in oncology depends on the ability of normal tissue to recover from the effects more effectively or efficiently than malignant tissue. Radiation causes both reversible and irreversible changes in normal tissue, and these effects are placed into two categories: acute effects (apparent during or shortly after the radiation course) and long-term effects (apparent from 6 months to years after completion of therapy). Radiation effects may not be initially apparent except by careful chemical or microscopic study. Indeed, the effects may not be apparent for many years or may manifest only in the offspring of the irradiated organism. The accepted position regarding radiation exposure is that incidental environmental radiation, diagnostic tests, and therapeutic radiation can all be detrimental. Although in many cases the chance of injury from diagnostic or environmental radiation is slight, the possibility of damage from a known exposure must always be weighed against the importance of the information to be gained or the effect desired. Incidental exposure must be avoided through control of environmental hazards whenever possible by following the ALARA (as low as reasonably achievable) principle.
Radiation Source | Average Annual Whole-Body Dose (Millirem/Year) |
---|---|
Natural: cosmic | 29 |
Terrestrial | 29 |
Radon | 200 |
Internal (e.g., 40 K, 14 C) | 40 |
Human made (diagnostic radiography, nuclear medicine, consumer products such as smoke detectors) | 64 |
All others (fallout, air travel, occupational) | 2 |
Average annual total | 360 |
Tobacco smokers | add ∼280 |
Activity | Typical Radiation Dose |
---|---|
Smoking | 280 mrem/year |
Chest radiography | 8 mrem/single radiograph |
Drinking water | 5 mrem/year |
Cross-country airplane trip | 5 mrem/year |
There are many forms and sources of radiation, including natural isotopes and human-made radiation. The natural radiation emissions (gamma and beta rays) of isotopes such as iridium, iodine, and cesium are used for therapeutic purposes in many human malignancies ( Table 22.4 ). In addition, during the past several decades, increasingly sophisticated machines have been manufactured to produce high-intensity, directed radiation to treat both malignant and benign conditions. Modern machines emit energies greater than 1 million electron volts (1 MeV) and are termed supervoltage or megavoltage machines ( Table 22.5 ). The most commonly available are called linear accelerators (linacs). Even these have recently become more sophisticated to allow increasingly precise delivery of radiation in the form of intensity-modulated radiation therapy (IMRT) and stereotactic body radiotherapy (SBRT). The newest generation models allow for real-time computed tomography (CT) for position verification, termed image-guided radiation therapy, or IGRT. Image guidance is also more commonly used in brachytherapy, which historically used only two-dimensional imaging. With the advent of CT- and magnetic resonance imaging (MRI)–compatible brachytherapy applicators, imaging modalities are used to more precisely direct the radiation to the tissues at risk while simultaneously avoiding normal, uninvolved tissues. The acceleration in technology has paralleled the advances in computer technology. In addition, simulations that combine radiation delivery planning with positron emission tomography (PET) and MRI are currently being used at several centers.
Element | Isotope | Emax (MeV) | T 1/2 | Clinical Use |
---|---|---|---|---|
Radium | 236 Ra | 3.26 | 1600 years | Historical |
Cesium | 137 Cs | 0.514, 1.17 | 30 years | Temporary intracavitary implants |
Iridium | 192 Ir | 0.38 ave | 74.2 days | Temporary interstitial implants and source for high dose rate machine |
Iodine | 125 I | 0.028 ave | 60.2 days | Permanent interstitial implants |
Phosphorus | 32 P | None | 14.3 days | Permanent intracavitary placement |
Palladium | 103 Pd | 20 kV | 17 days | Permanent prostate seed implant |
Modality | Voltage | Source |
---|---|---|
Low voltage (superficial) | 85–150 keV | X-ray |
Medium voltage (orthovoltage) | 180–400 keV | X-ray |
Supervoltage | 500 keV–8 MeV | Linear accelerator, 60 Co machine |
Megavoltage | Above supervoltage energy | Betatron, linear accelerator |
Radiation units
Those in training and in practice must familiarize themselves with the international system (SI) of weights and measures used today. The SI units and appropriate conversions are shown in Table 22.6 .
Quantity | Historical Unit | International System (SI) Unit | Conversion Factor |
---|---|---|---|
Exposure | R | C/kg | 2.58 × 10 −4 C/kg/R |
Absorbed dose | Rad | Gray (Gy) | 10 −2 Gy/rad |
Dose equivalent (used in radiation protection) | Rem | Sievert (Sv) | 10 −2 Sv/rem |
Activity | Curie (Ci) | Becquerel (Bq) | 3.7 × 10 10 Bq/Ci |
When a radiation exposure occurs, the resultant ionizations deposit energy in the air. If a patient lies in the path of the beam, energy will be deposited in the patient. This deposition of energy by radiation exposure is called r adiation- a bsorbed d ose, measured in the acronym rad. One rad is equivalent to the deposition of 100 ergs of energy into each gram of the irradiated object. The SI unit of radiation absorbed dose is the gray (Gy), and 1 Gy = 1 cGy = 100 rads = 1 J/kg. The erg and joule are units of energy.
Radiation physics
Energy deposition
Radiation energy is deposited into the tissue in one of two ways: direct or indirect ionization. Ionization is when an outer shell electron is stripped from an atom, leaving a positive charge. Direct ionization is the predominant mechanism of action of particles that possess charge, but photons can also cause direct ionization. Examples of directly ionizing particles include particulate radiation such as protons and neutrons. Indirect ionization produces free radicals of other molecules, namely water, which diffuse and damage critical targets ( Fig. 22.1 ). A free radical has an unpaired outer shell electron that is chemically unstable and very reactive. The hydroxyl free radical (made by the lysis of H 2 O) diffuses only about 1 nm and breaks the chemical bonds in cellular proteins and other key substances such as DNA. Either form of energy deposition results in ionization of target molecules. Scientists estimate that about two-thirds of cell biologic damage is from indirect action by ionizing radiation, from sources such as x-rays and gamma rays.
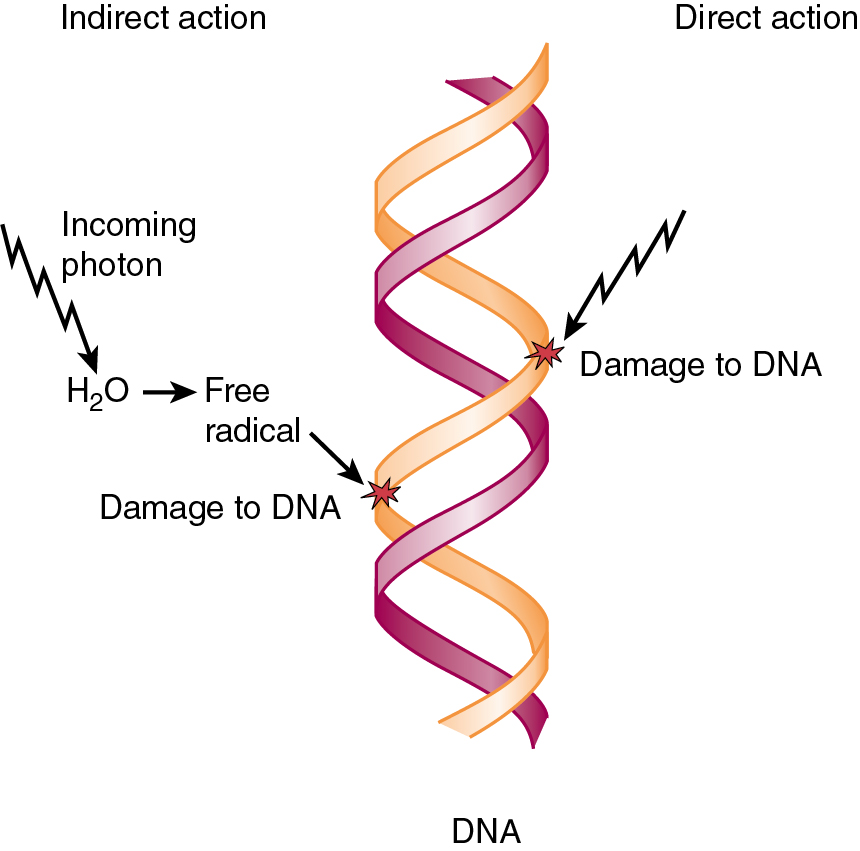
The electrons generated by penetrating photons lose their energy slowly, resulting in a low energy deposition along the electron track, or low linear energy transfer (LET; defined as energy transferred per unit length of track, or keV/μm). High LET radiations (neutrons, negative p mesons, or alpha particles) deposit more energy per unit length, are more commonly directly ionizing, and are less susceptible to perturbations such as oxygen tension. This means that for the same reaction produced in healthy cells by all radiation modalities, high LET radiation has a higher probability (1.5 to 2.5 times that of x-rays) of killing those cells in less than ideal circumstances (i.e., hypoxic tumor cells, cells in a resistant part of the cell cycle).
Sources of radiation
Gamma rays are the photons emitted from the atomic nuclear decay of radioactive isotopes—for example, 137 Cs (cesium) or 60 Co (cobalt). X-rays are photons electrically generated by bombarding a target such as tungsten with electrons (how a linear accelerator works). When these fast-moving electrons approach the tungsten nuclear field, they are attracted to the nucleus and thus veer from their original path. This change in direction causes deceleration and kinetic energy is converted to x-rays in the form of bremsstrahlung photons. These emitted x-rays, or photons, vary in energy from zero to a maximum determined by the kinetic energy of the bombarding electrons. Machines such as the betatron and linear accelerator generate electrons with high kinetic energy and thus produce high-energy x-rays. In addition to bremsstrahlung photons, characteristic photons are also produced as atoms seek to fill electron orbital vacancies (see later discussion). Gamma rays and x-rays are collectively called photons , and what is of medical importance is the energy and delivery of the photon, not the source.
Photon interactions
The interaction of photons with matter is accomplished through six mechanisms: (1) Compton scattering, (2) photoelectric absorption, (3) pair production, (4) triplet production, (5) photodisintegration, and (6) coherent scattering (no energy transfer). The Compton effect is the major interaction of photons in tissue used in modern radiotherapy ( Fig. 22.2 ). When the photon from the linear accelerator interacts with outer orbital atomic electrons, part of the photon energy transfers to the electron as kinetic energy. The photon is deflected with reduced energy. The ejected electron is propelled forward and sets up a cascade of increasing energy deposition by displacing more electrons. As a result of this initiation and subsequent buildup effect, megavoltage photon beams have a skin-sparing effect not seen in older machines and therefore produce less superficial tissue change.
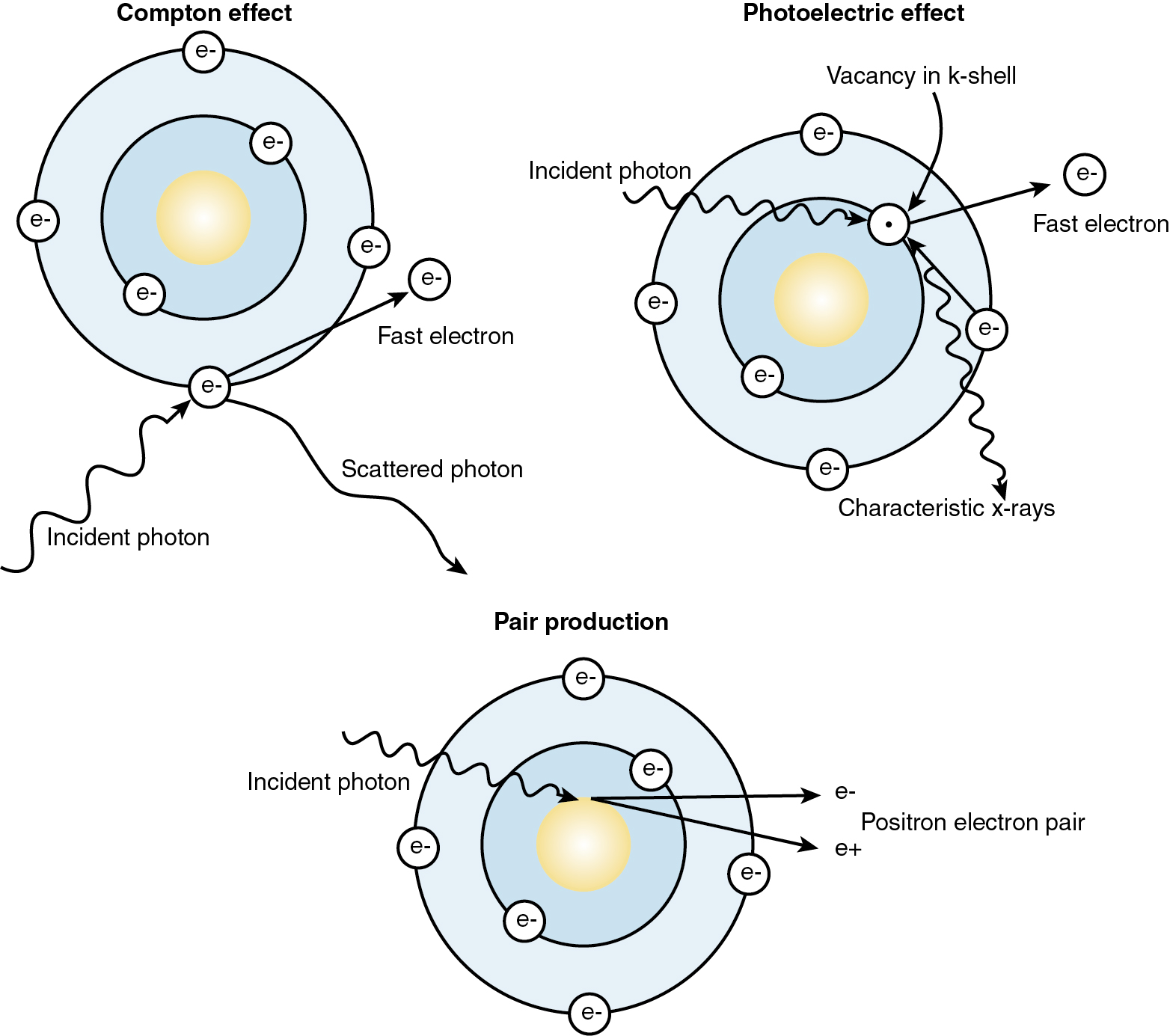
The photoelectric effect is seen at lower energies. This effect is the most important in diagnostic radiology. In this interaction, the incident photon is absorbed completely by an inner shell electron. The inner shell electron is ejected with kinetic energy equal to the incident photon energy less the electron-binding energy. An outer shell electron then drops into the vacancy. As this electron changes orbit, its energy is reduced, and the excess energy is given off in the form of a photon, called a “characteristic photon.”
In pair production, photon energies greater than 1.02 MeV interact with the strong electric field of the nucleus and lose all incident energy. The incident photon energy is converted into matter in the form of a positron–electron pair. If this happens in the field of an orbital electron, three particles are produced in the interaction, and the interaction is called triplet production.
Last, in photodisintegration, the high-energy photon enters the nucleus and ejects a neutron, proton, or alpha particle. This is important for shielding considerations in linear accelerators that operate at energies above 15 MeV.
Radioactive decay
Naturally radioactive substances decay to more stable substances by several methods: (1) beta decay ( 32 P, 18 F), (2) electron capture ( 125 I), (3) alpha decay ( 226 Ra), and (4) isomeric transition (gamma emission and internal conversion). “Decay” is the manner in which an isotope releases, or gains, matter or energy to become a more stable substance. The rate of decay of a radionuclide is exponential and is termed the “activity.” The old unit for activity was the Curie (Ci). The SI unit is the becquerel (Bq) defined by 1 Bq = 1 dps (disintegration per second). The half-life (T 1/2 ) is the time required to disintegrate to half the original activity. T 1/2 of commonly used radioactive substances is in Table 22.4 .
137 Cs (cesium-137), used in low dose rate (LDR) applications, is a byproduct of the fission process in a nuclear reactor and decays via beta and gamma emission. In beta decay, a neutron from the nucleus converts into a proton (positively charged) and an electron. Again, to increase stability, a photon is released, and the 127 Cs becomes the more stable 137 Ba ( Fig. 22.3 ). 137 Cs decays approximately 2%/year.
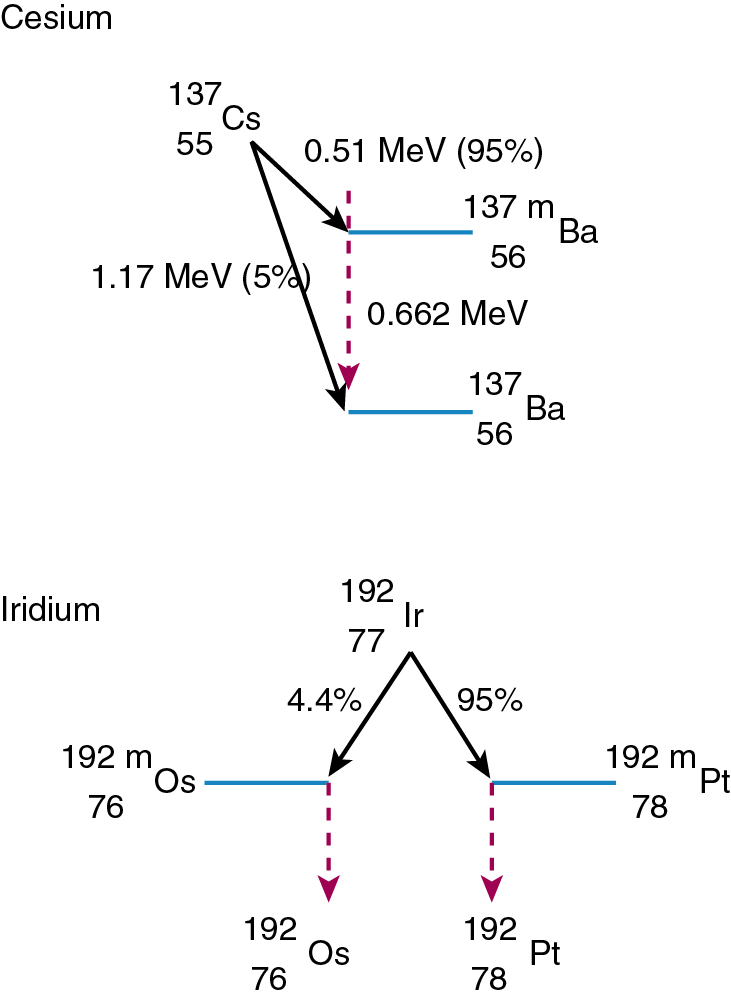
192 Ir (iridium 192) is also produced in a nuclear reactor and decays via beta decay (see earlier) and electron capture. In electron capture, the nucleus “captures” an orbital electron and converts a proton into a neutron. 192 Ir then becomes the excitable 192 m Pt and 192 m Os, which release gamma rays to stabilize. 192 Ir is the primary isotope used for high dose rate (HDR) applications and interstitial implants. It decays approximately 1%/day.
Inverse square law
Another important physics concept in radiotherapy is the inverse square law. This law states that the dose of radiation at a given point is inversely proportional to the square of the distance from the source of radiation (dose ∝ 1/distance 2 ). This rapid decrease in dose is very important in brachytherapy. It underscores why the bladder and rectum can be relatively protected from the high intracavitary doses of radiation, especially with good vaginal packing to maximize the distance from the source to the normal organ. It also underscores why good geometry is critical when performing implants. Finally, it explains the reasoning behind standing at the door (increasing the distance) while conversing with a brachytherapy patient. As a simplistic example, consider the following. The dose at point A is 4. Two feet away the dose would be 4 divided by the square of the distance (2 2 ) or 4/4 = 1. Another 2 feet away, the dose would be 1/4 (4/4 2 = 4/16 = 1/4).
Depth dose characteristics of radiation
The last physics concept to master is variation in radiation beam characteristics based on energy. To that end, it is important to realize that the energy and penetrating power of ionizing radiation increase as the photon frequency increases and wavelength decreases. In addition, as energy increases, the depth of maximum dose increases (D max ; remember the buildup effect discussed earlier). For low energies, such as 250 keV, the maximum dose is at the skin surface. For a 4-MeV (4 million electron volts) accelerator, the D max is approximately 1 cm; for 6 MeV, D max is at 1.5 cm; for 22 MeV, D max is at 3.5 cm; and so on. Knowing this, one can see why higher energy beams are more suited to treat deeply seated tumors, such as in the uterine or cervix. These higher energy beams differentially spare more superficial tissues. Depths of maximum dose curves and isodose distributions (areas receiving similar dose) for various energies are shown in Fig. 22.4 .
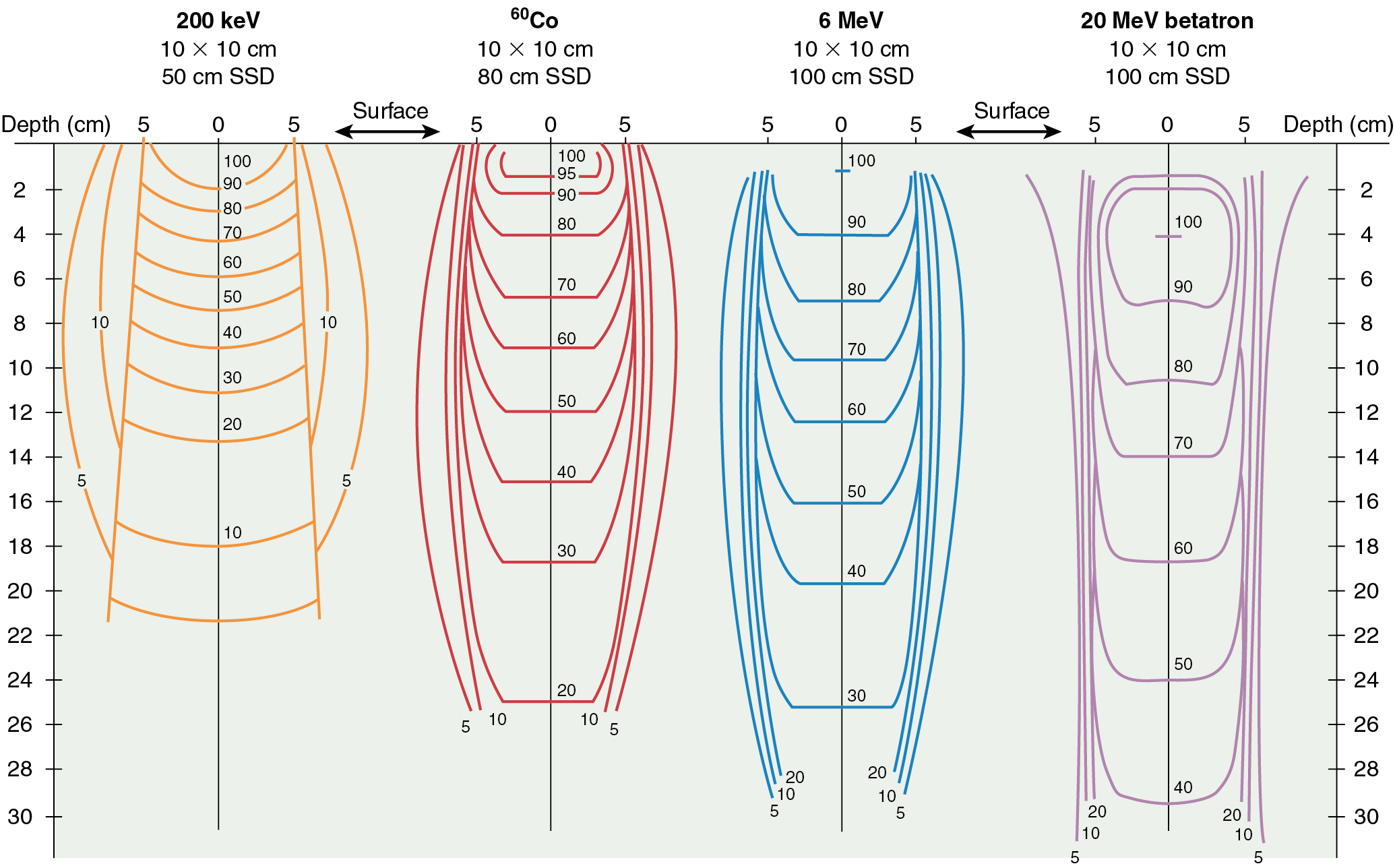
The reduced effect on skin of supervoltage radiation, compared with orthovoltage (keV range) radiation, is based on a physical characteristic of radiation. With higher energy, forward movement of the energy cascade (in the direction of the primary beam) is greater with reduced lateral scattering. As the energy increases, it becomes more penetrating, and the photons and resultant liberated electrons travel a greater distance into the absorbing material. Therefore, the percentage of radiation at any specific depth, compared with the surface dose, increases as the energy increases.
In summary, above the energy of 400 to 800 keV, the advantages to higher energy photons are less damage to the skin at the portal of entry, greater radiation at depth relative to the surface, and reduced lateral scatter of radiation in the tissues. In addition, there is less photoelectric effect at higher energies and therefore less absorption in bone.
For particle-based therapy, such as electrons and photons, the percent depth dose characteristics follow different principles. For electrons, the percent of dose deposited at the skin surface is much higher, on the order of 80% to 100%. Modern high-energy linear accelerators provide electron beam energies in the range of 4 MeV to 25 MeV. As the energy of the electron beam increases, the percent dose deposited at the surface also increases, in contrast to photons where the surface dose decreases as the beam energy increases. Also as the electron beam energy increases, the depth at which the maximum dose is deposited also increases (approximately 2 cm for 6 MeV and 6 cm for 18 MeV beam). However, unlike photons, the dose beyond the max dose depth levels off rapidly beyond that distance with a bremsstrahlung tail on the order of a few percent. Even for a higher energy 18 MeV electron beam, the dose at 10 cm has already fallen below 5%.
Protons are heavy charged particles, approximately 800 times the mass of electrons. The large mass and the acceleration used results in a specific momentum that dissipates rapidly as the proton travels within tissue. As the protons lose energy, there are increased interactions with orbiting electrons which result in a sharp rise in energy deposition at the end of the path of the proton, termed the Bragg peak. Beyond the peak (point of maximum dose deposition), there is no further dose delivery; it is this feature of protons which provides a unique dosimetric advantage over photons and electrons. This Bragg peak can be positioned within the clinical target with superior sparing of the tissues beyond the target.
Radiobiology
The selective destruction of tissues forms the basis of therapeutic radiation. Neoplastic cells are preferentially killed by radiation compared with the surrounding normal tissues, primarily as a result of differences in repair capabilities. This differential radiosensitivity between normal and cancerous tissues determines in large part whether a radiated neoplasm is eradicated. The ratio that defines the dose necessary to effect tumor kill with the dose likely to cause normal tissue damage is the therapeutic ratio. Modern radiotherapy strives to maximize this ratio ( Fig. 22.5 ).
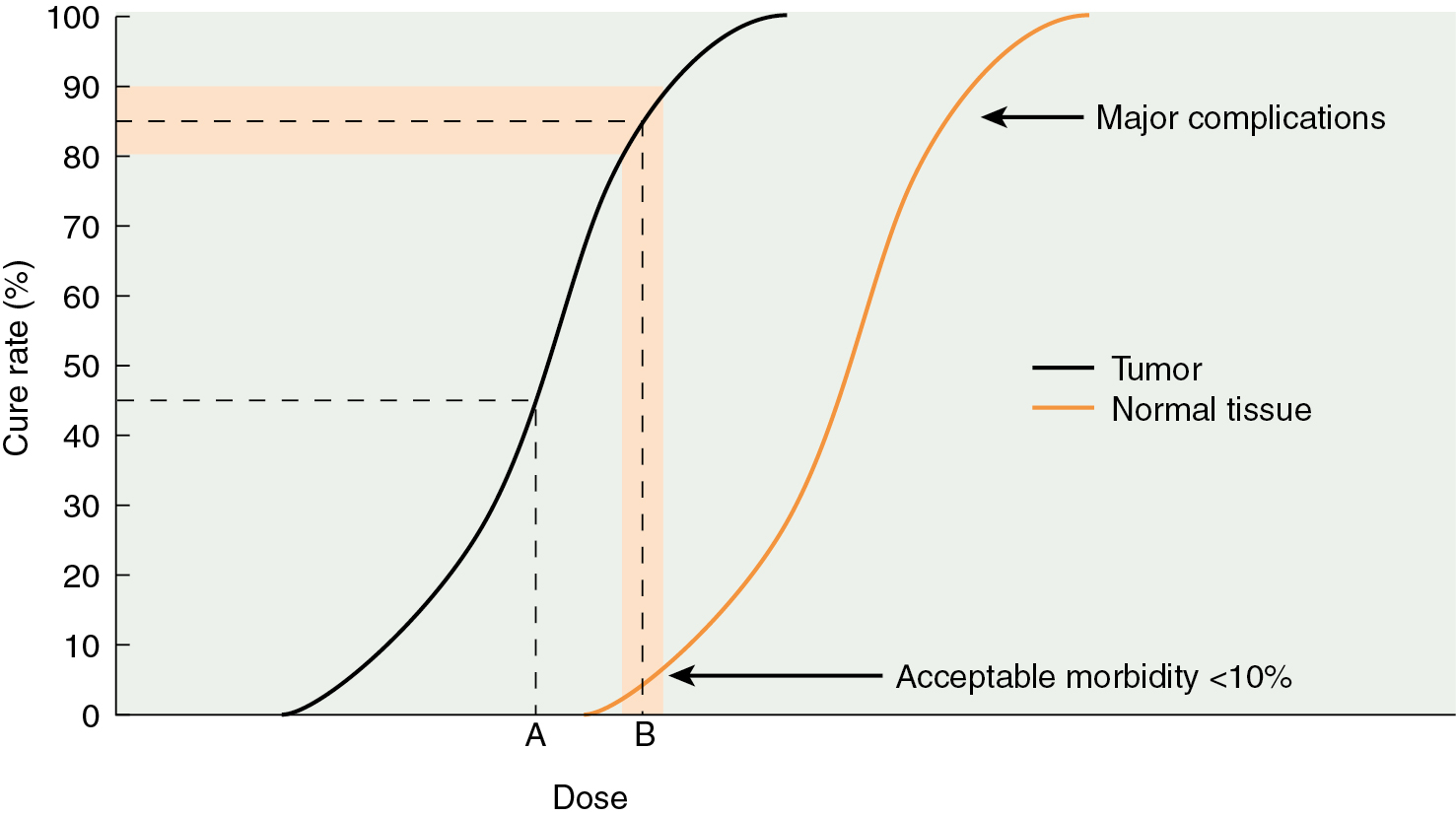
Structural changes
Deposition of radiation energy in the cell can lead to a variety of changes that alter normal function. Degradation, or breaking into smaller units, and cross-linking are examples of structural damage that can affect proteins, enzymes, and nucleic acids. The initial chemical change occurs in a fraction of a second and is rarely detected directly. Some changes are repaired almost immediately, but others can never be repaired. Although various morphologic and functional changes occur in irradiated cells, the bulk of direct and indirect evidence suggests that the biologic effects of radiation result principally from DNA damage. For example, it has been calculated that 1 million cGy is required to inactivate cell cytoplasmic enzyme systems, and doses of 1000 cGy are required to damage cell membranes. In contrast, chromosomal aberrations and mutations can be produced by very low doses of radiation. Because only a few hundred cGy are needed to produce a high degree of lethality in cell tissue culture, it seems logical that nuclear changes are responsible for cell death.
Radiation-induced DNA damage destroys the cell by one of several mechanisms. It may stimulate cell apoptosis, called interphase death, which is visible by several methods, including light microscopy and Western blot. Radiation may also cause mitotic death. In this form of damage, the cell outwardly appears normal but is sufficiently damaged so that cell division is not possible. Actual death of the cell does not occur, however, until cell division is attempted. This is why tumors may continue to regress after completion of radiation.
A variety of DNA lesions are produced by radiation including base damage, DNA–protein crosslinking, single-strand breaks, and double-strand breaks (DSBs). Repairing even a single nucleotide requires many genes and a series of steps. The most toxic cellular lesion inflicted by ionizing radiation is the DSB. In fact, the proficiency of repair of DSB correlates well with radiation-induced lethality. The repair of DSB can occur by several mechanisms. Religation of complementary DSB ends, homologous recombination (HR), is efficient, and cells can usually survive. The Mre11 protein is attracted when a DSB occurs and may be the first sensor of the break and primarily involved in repair through nuclease activity, unwinding DNA, or removing hairpin loops. When the ends are not complementary, nonhomologous end joining (NHEJ) is used and can be complicated by small DNA deletions or insertions. These errors in turn can lead to cell death or mutation. Some of the necessary proteins for NHEJ are Ku70, Ku80, DNA ligase IV, and XRCC4. The Ku70/Ku80 complex binds to the DSB and unwinds the strand while the DNA ligase IV/XRCC4 complex repairs DSBs. Mammalian cells seem to repair more by NHEJ than HR.
Radiosensitivity
Radiosensitivity is the response of the tumor to irradiation that can be measured by the extent of regression, rapidity of response, and response durability. Radiosensitivity depends on several factors. These factors include the ability to repair damage, hypoxia, cell cycle position, and growth fraction. In addition, the volume of the initial tumor has been demonstrated to influence the ability to eradicate tumors.
Understanding that radiosensitivity and radiocurability are not identical in meaning is essential. Relatively radioresistant tumors accessible to high-dose local radiation therapy can be cured, but radiosensitive tumors that are widely metastatic can only be controlled locally. An excellent example of a relatively radioresistant tumor is squamous cell carcinoma of the cervix; however, this malignancy remains one of the most curable tumors in humans because of its accessibility to high-dose irradiation and the relatively radioresistant nature of the hosting normal tissues (e.g., cervix and vagina). The ability to place iridium or cesium in juxtaposition to the malignancy within dose ranges tolerable to the surrounding normal tissue is the key to success.
Many attempts have been made to develop an assay to predict tumor radiosensitivity. However, no assay yet developed accurately predicts the outcome in a given tumor. This is likely secondary to tumors containing mixed cell populations with differing sensitivities to chemotherapy and radiation. Sensitive cells are eliminated, but resistant cells continue to grow. This explains why many tumors initially respond to therapy but are ultimately incurable.
In vitro models have been developed to predict and study tumor cell radiosensitivity. Cellular radiosensitivity is generally quantified by measuring the loss of reproductive capacity, which can be plotted in a survival curve. Survival curves are characterized by an initial slope, α, and the terminal slope, β. α represents irreparable damage to the cell, and β represents the repairable damage ( Fig. 22.6 ). The α/β ratio is the dose at which the contribution from alpha equals the contribution from beta and is a measure of radiosensitivity. Large ratios are seen with rapidly dividing cells and help predict the response of tumors and the early effects of radiation. Low ratios characterize late responding tissues.
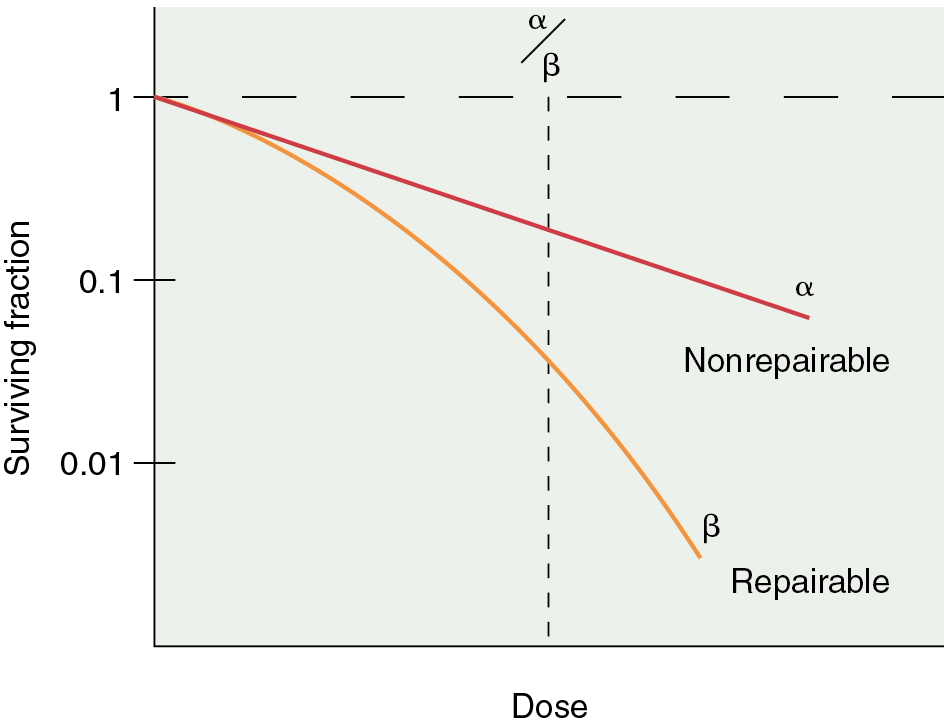
In addition, the size of the shoulder (Dq) on a survival curve relays valuable information because it represents the magnitude of repair of sublethal damage (SLD). Broad shoulders have small α/β ratios and good repair of SLD. This repair of SLD takes several hours (2 to 6 hours) to complete. The ability of cells to repair SLD has led to the use of fractionated doses in clinical radiation therapy, in which differential capacities between normal tissue and tumor to repair a sublethal injury can be exploited ( Fig. 22.7 ). This differential repair capacity also forms the basis for accelerated fractionation or hyperfractionation in which the dose is given twice a day. This approach works very well with rapidly growing tumors. Treated twice a day, the normal tissues have sufficient time to repair, but the tumor tissues, which are less organized, efficient, and accurate with repair, are differentially killed. Some cells have almost no shoulder, indicating a limited ability to repair SLD, and these cells can be eradicated with relatively low doses of radiation. For example, dysgerminomas are highly curable with relatively low doses of radiation (20 to 30 Gy) compared with cervical cancer tumors, which may require more than 70 Gy to obtain cure.
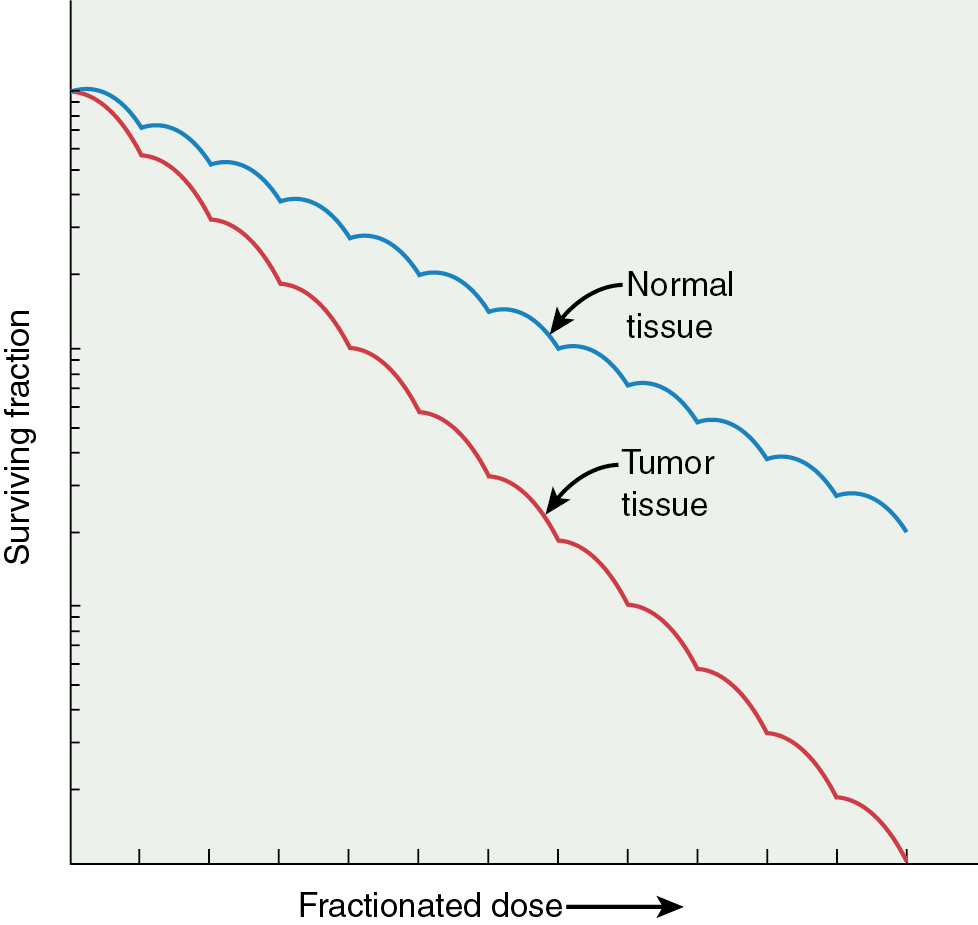
Radiosensitivity of cells is also dependent on the availability of oxygen. As radiation enters the cell, it interacts with organic molecules (DNA). This molecule may repair itself unless an oxygen molecule becomes attached, thereby preventing repair mechanisms from proceeding. The addition of O 2 will enhance low LET radiation (photons) but does not similarly affect high LET radiation. The ability of oxygen to enhance radiation is measured by the oxygen enhancement ratio (OER). The OER is calculated by dividing the dose of XRT without O 2 for a given effect by the dose of XRT with O 2 for the same effect. It takes at least 2% (17 mm Hg) tissue O 2 to see the full oxygen effect.
Tumor cells with potentially unlimited capacity for growth are inhibited as they outgrow the blood supply. In fact, tumors above 200 μm have necrotic cores secondary to a limited diffusion distance for oxygen. Oxygen may effectively penetrate approximately 70 μm from the blood vessel ( Fig. 22.8 ). Beyond this distance, tumor cells become deficient and enter a noncycling phase. They may become hypoxic or even anoxic and necrotic. This is important from a radiobiologic standpoint because noncycling cells exhibit a greater capacity to repair radiation injury and are considered radioresistant. Thus large tumors can be difficult to control with radiation therapy, not only because there is a greater number of cells to sterilize but also because a proportion of these cells are hypoxic, noncycling, and radioresistant. As a clinical example, experience notes that exophytic friable cervical lesions that hemorrhage easily on contact respond better and more quickly than infiltrative lesions. The blood supply and oxygenation vary considerably between these two lesions, with the friable lesion better vascularized and oxygenated and therefore more radiosensitive. Unfortunately, attempts to overcome this differential sensitivity by mechanisms such as hyperbaric oxygen and radiosensitizers have not proven successful.
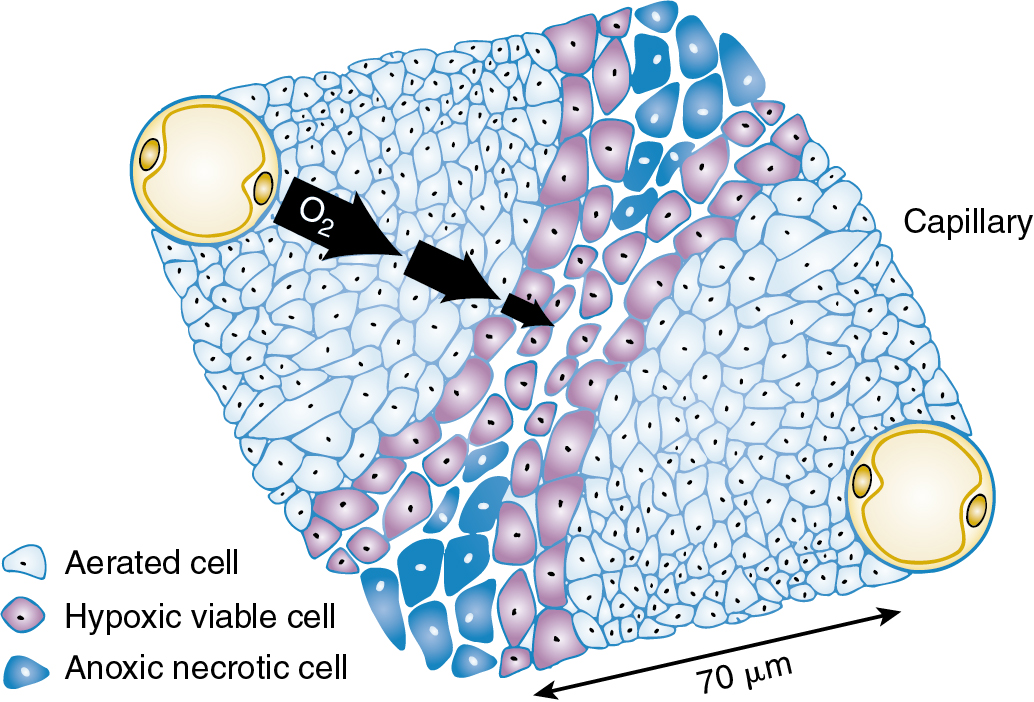
Another factor important in radiosensitivity is the proportion of mitotic or clonogenic cells in the tumor. Cycling cells are more radiosensitive. In fact, mitotic counts have been shown to correlate with the prognosis in many tumors. The position in the cell cycle is also important ( Fig. 22.9 ). Cells in late G2 and mitosis (M-phase) are the most sensitive to radiation, and cells in late synthesis (S-phase) are the most resistant ( Fig. 22.10 ). This is exploited with chemotherapies such as paclitaxel, which arrests cells in mitosis and is a profound radiation sensitizer.
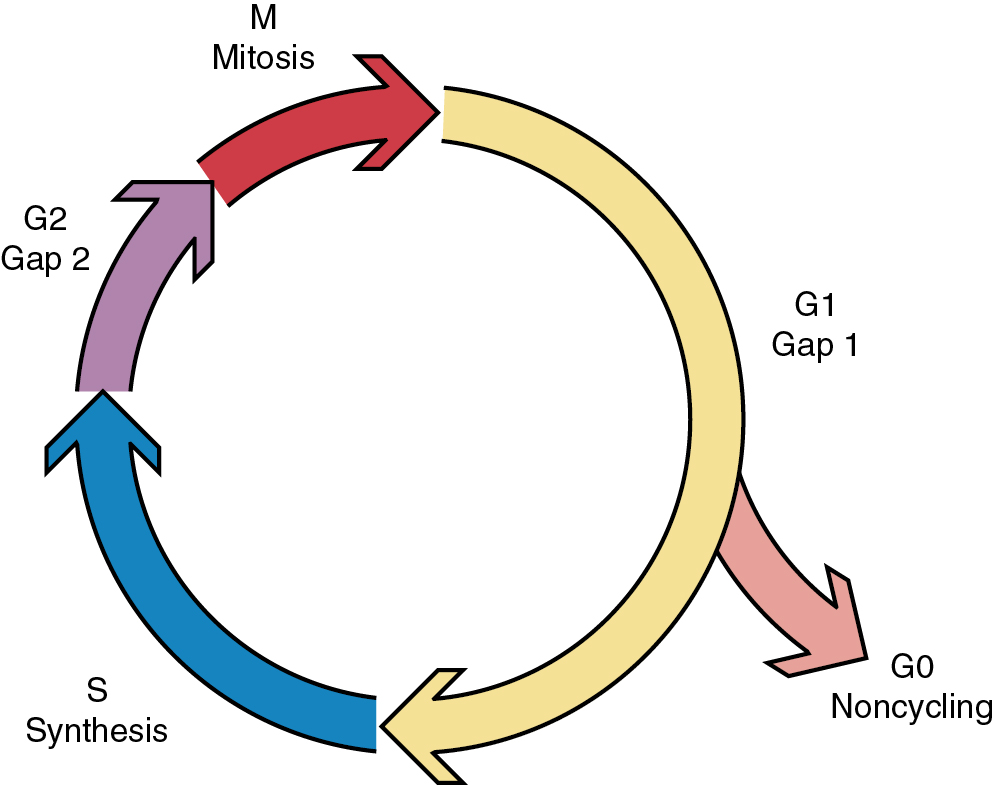
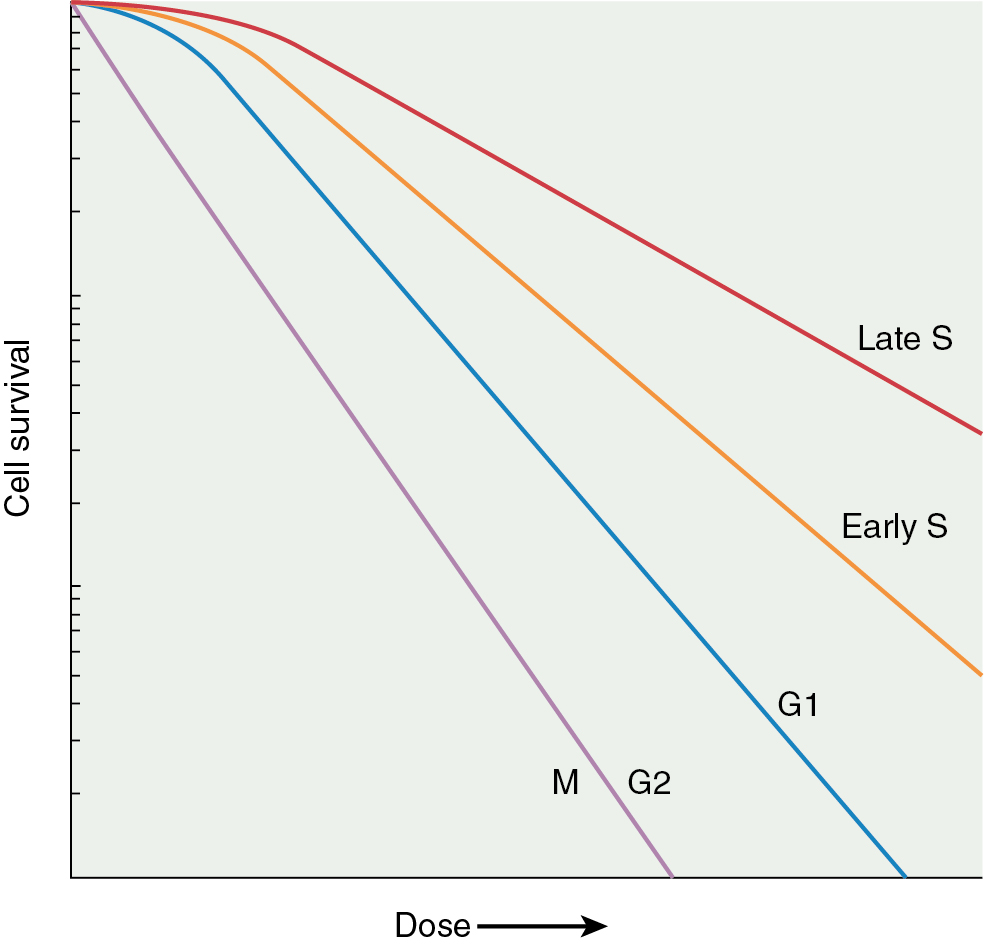
Finally, the initial tumor volume greatly influences the ability to sterilize a site. Generally, the smaller the tumor volume, the less radiation is required to destroy all cells. As the volume increases, the dose to obtain local control is increased. The concept of treating with “shrinking” fields is to serially reduce the size of the radiation portals to give a higher dose of radiation therapy to the central portion of the tumor, where presumably more hypoxic, radioresistant cells are present. With more modern technology the concept of shrinking fields has been replaced by a simultaneous integrated boost (SIB) which uses IMRT to deliver higher doses centrally with lower doses along the periphery of the tumor adjacent to critical normal structures.
Historically, clinicians attempted to correlate radiation tumor response and local tumor control. Generally, the faster and more complete the tumor response at the completion of the therapy, the greater long-term control, assuming that no distant metastasis occurs. Although this is not uniformly true, it has been shown in cervical cancer that there is a good correlation between local tumor control and complete or partial regression of the cancer at the completion of radiation. In 2004, Grigsby and associates established posttreatment PET tumor response (which measures glucose metabolism) correlates with survival, which has been confirmed by several subsequent studies.
Another factor limiting radiocurability is the normal tissue toxicity with increasing radiation doses. Normal tissue effects depend on total dose, dose fraction size, volume, and inherent tissue radiosensitivity. The ultimate goal is to obtain maximal tumor control with minimal side effects. Combining radiation therapy with surgery or chemotherapy decreases normal tissue tolerance to a given dose. Well-established treatment fields or tumor margins, total dose, and fractionation schemes have been identified but cannot remain static. Variations are being investigated to increase curability and decrease complications, including new chemotherapeutic radiosensitizers, technological advances in the delivery of radiation such as IMRT, and radioprotectors such as Ethyol.
Radiobiology of ablative radiotherapy
As discussed previously conventional radiation therapy primarily relies on fractionation, or delivering lethal doses of radiation in several small fractions, to maximize the therapeutic ratio by multiple mechanisms: repair of SLD in the normal tissues between each fraction, and for malignant tissue this allows redistribution of cells to more vulnerable phases within the cell cycle, and reoxygenation of the surviving cells to increase percent cell death with subsequent fractions. SBRT, also knowns as stereotactic ablative therapy (SABR), differs from conventional radiation therapy by delivering lethal doses in one, or up to 5 large fractions. This method of delivery does not discriminate between normal or malignant tissue and results in cell death confined to a highly conformal target which is typically the tumor itself with very small margin of 1 to 3 mm. The mechanism of cell death resulting in enhanced anti-tumor effect is postulated to occur through multiple biologic effects. First, we know that ablative doses of radiation cause cell death through an increased number of direct double-strand DNA breaks as compared to conventional doses of 1.8 to 2.0 Gy. Additionally multiple studies have demonstrated that at doses of 10 Gy and above, secondary cell death occurs as a result of vascular damage and increasing tumor hypoxia. Finally, we have data to suggest that the large amount of tumor antigens and immune factors released as a result of direct and indirect tumor cell death after higher ablative doses increases the body’s inherent anti-tumor immune response. This may result in the phenomena known as the abscopal effect whereby metastases treated with ablative doses of radiation may result in the regression of disease in other sites of the body not previously treated with radiation.
Radiosensitizers, hypoxic cell sensitizers, and radioprotectors
Radiation sensitizers and protectors are being actively investigated to increase the effectiveness of radiation or allow dose escalation while minimizing side effects. Radiation sensitizers increase the ability of radiation to cause permanent, lethal damage. This therapeutic gain may be from a variety of mechanisms, including selective uptake, targeting, or activation. To be effective, sensitizers must have acceptable normal tissue side effects. Known radiation sensitizers include chemotherapeutic agents such as cisplatin, 5-fluorouracil, paclitaxel, Adriamycin, and gemcitabine. Hypoxic cell sensitizers include the imidazole drugs such as misonidazole and bioreductive agents such as tirapazamine. Endogenous sulfhydryl compounds and Ethyol confer radioprotection to normal tissues by scavenging oxygen free radicals.
The advantages afforded by chemoradiation may arise from several mechanisms. Some agents (e.g., cisplatin) inhibit the repair of radiation damage, but others (e.g., paclitaxel) block cells in a radiosensitive cell cycle phase. Adriamycin, docetaxel, and paclitaxel have unique effects when combined serially with radiation because “radiation recall” is an uncommon but distressing phenomenon. After a course of radiation, the normal tissue sensitization can be “recalled” when the chemotherapy is initiated—this phenomenon is a reemergence of a prior radiation burn that conforms exactly to the prior radiation portal. The mechanism of this is unknown but well described for these chemotherapies.
The hypoxic cell sensitizers that have been studied to date, including the nitroimidazoles and the bioreductive agent tirapazamine, have demonstrated significant toxicity and little clinical utility. Ongoing research into these classes of drugs will be necessary to exploit this theoretical avenue of tumor sensitization.
Interestingly there are two oxygen modifiers of endocrine origin that are currently being explored in the clinical trial setting. Metformin is thought to have a radiosensitizing effect through the reduction of tumor hypoxia based on pre-clinical studies. It is currently being studied on a phase II trial with the administration of metformin 1 week before and during concurrent cisplatin-based chemoradiation for patients with International Federation of Gynecology and Obstetrics (FIGO) stage IB2-IVA cervical cancer. A (18) F-Fluoroazomycin arabinoside (FAZA)-PET scan will be used to assess the change in fractional hypoxic volume of the tumor at baseline and after the 1-week lead-in dose of metformin. Endostar, a recombinant human endostatin, is another hypoxia modifier currently under investigation. It is an anti-angiogenic agent thought to alter the metabolic environment of the tumor based on mouse models. There are currently two phase II trials in development, both looking at the addition of Endostar to standard chemoradiation, one specifically for patients with high-risk early-stage cervical cancer requiring adjuvant chemoradiation, and the other for patients with locally advanced cervical cancer being treated with chemoradiation in the definitive setting.
Radioprotection has been proved in several sites with the use of Ethyol. Amifostine (Ethyol; WR-2721) is an organic thiophosphate extensively studied by the Walter Reed Army Institute of Research. Normal tissues noted to be protected included the salivary glands, bone marrow, immune system, skin, oral mucosa, esophagus, intestinal mucosa, kidney, and testes. It is dephosphorylated to the active metabolite (WR-1065) in the tissues. It is believed that tissue versus tumor alkaline phosphatase concentration variations provide the differential protective effect. In addition, tissue pH differences afford selective tissue versus tumor uptake of Ethyol. When given intravenously, the plasma half-life is less than 10 minutes, but there is prolonged retention of the drug in normal tissue. In the first 30 minutes, drug uptake into normal tissues has been shown to be 100 times that of tumor tissues. When inside the cell, the metabolite scavenges oxygen free radicals and provides an alternative target for alkylating agents, such as cisplatin.
There have been numerous studies of the protective effect of Ethyol with no reported decrease in antitumor efficacy. A phase III trial in head and neck cancer unequivocally established its role in salivary gland protection, confirming earlier studies. Other clinical trials have shown Ethyol protects from cisplatin-induced renal, neurologic, and bone marrow toxicity. Liu published a phase III randomized trial in rectal cancer patients treated with pelvic radiation with and without Ethyol (340 mg/m 2 ). The toxicity in the mucous membranes and genitourinary (GU) and gastrointestinal (GI) tracts was reduced by 50% in the Ethyol arm. Moderate to severe late toxicities were seen in 14% of those treated without Ethyol compared with zero in the Ethyol-treated patients.
Athanassiou and colleagues randomly assigned 205 patients with pelvic radiation plus or minus Ethyol and demonstrated a significant decrease in bladder and GI toxicity. Complete responses to therapy and median survival were not significantly different between the arms. The RTOG (Radiation Therapy Oncology Group 0116) completed a two-arm trial using Ethyol with chemoradiation for pelvic and paraaortic radiation with concomitant cisplatin in cervical carcinoma. Arm 1 consisted of cisplatin chemotherapy concomitant with paraaortic radiation and demonstrated significant acute and late toxicity. Arm 2 again demonstrated significant acute and long-term toxicity, with no significant benefit demonstrated with the addition of Ethyol, although compliance to the medication was disappointing. Ethyol has been found to be useful in reducing early and late toxicity with hypofractionated accelerated conformal radiation by Koukourakis. Toxicities with intravenous administration include hypotension and nausea. Data on subcutaneous administration demonstrate less hypotension but treatable nausea and skin effects persist. There have been rare reports of Stevens-Johnson syndrome.
Genetic effects
It is not possible to generalize and assign a specific mutation rate to a given radiation dose. Gene loci differ greatly in their mutability, and the random damage exerted by irradiation on any particular chromosome makes predictability impossible. The mitotic stage, cell type, sex, species, and dose rate influence the mutation rate as studied in lower animals and bacteria. Data from lower animals are difficult to extrapolate to humans, and therefore prediction of mutation rates cannot be expected from the evidence that has been accumulated from various types of radiation exposure. Direct evidence of radiation-induced mutation in humans is lacking, although there is strong anecdotal evidence for carcinogenesis in those exposed to radiation. A few examples are excess skin cancers seen in those exposed to x-rays before safety standards were established, lung cancer in pitchblende miners, bone tumors in radium dial painters, and liver tumors in those exposed to Thorotrast contrast. The largest groups of humans available for study are survivors and descendants of those exposed in Hiroshima and Nagasaki. Although there has been no detectable effect on the frequency of prenatal or neonatal deaths or on the frequency of malformations in subsequent generations, this does not mean that no hereditary effects were produced. The number of exposed parents was small, and dosages were so low that it would have been surprising if an increase in mutation had been detected in such a brief period. There has not been sufficient time for the several generations needed to reveal recessive damage.
Radiation does not produce new and unique mutations but increases the incidence of spontaneous mutations. Based largely on experiments in mice, it is estimated that the doubling dose (i.e., the dose that will double the spontaneous mutation rate) for humans is probably 100 cGy. Between 1% and 6% of spontaneous mutations in humans can be ascribed to background radiation. With the use of image intensifiers, improved radiographic film, and appropriate shielding to prevent scatter, it is possible to attain satisfactory radiographic visualization of internal structures with reduced exposure. The average dose of irradiation to the gonads of some common diagnostic techniques is given in Table 22.7 . The use of diagnostic radiographs and CT scans has increased and fueled concerns that toxic radiation effects may emerge, especially among children exposed to increased diagnostic medical irradiation. Current recommendations stress the use of ionizing radiation only when necessary and substitution of alternate sources of imaging, such as MRI, which relies on magnetic fields, when possible.
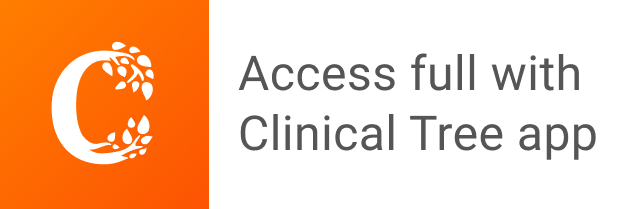