Keywords
Transkingdom RNAi (tkRNAi), bacteria-mediated RNAi (bmRNAi), RNA-based therapeutics, RNA interference (RNAi), Cancer, Bacteria
Introduction
During approximately the past three decades, researchers have been searching for new and improved methods to efficiently influence gene expression levels and to selectively switch off specific genes in diseased tissues . The most promising of these approaches focused on the design of RNA-based therapeutics. Starting in the 1980s, DNA- and RNA-based antisense oligonucleotides began to be developed. Various modifications in nucleotide chemistries were analyzed in detail in different model systems both in vitro and in vivo . In the 1990s, ribozymes became the focus of research. Ribozymes showing both antisense and catalytic properties were successfully applied for specific knockdown of target genes. In particular, hammerhead ribozymes were demonstrated to selectively alter the expression of various cancer-associated genes in human cell lines as well as in tumors grown in mice. However, insufficient efficacy of delivery for these therapeutic RNA molecules limited their clinical exploitation. In the 2000s, advances revealed new opportunities for the development of RNA therapeutics, particularly those based on the RNA interference (RNAi) mechanism.
Due to the enormous impact, the initial characterization of the RNAi phenomenon in the nematode Caenorhabditis elegans by Fire et al . led to these researchers being awarded the Nobel Prize in Physiology in 2006 . This landmark discovery identified double-stranded RNA (dsRNA) as a sequence-specific mRNA-interfering species. Mechanistically, RNAi is now well understood, and numerous review articles are available that provide detailed information of the basic biochemistry involved . Briefly, the first step in this naturally occurring biologic mechanism is initiated by processing of long regulatory dsRNA such as noncoding microRNA (miRNA) into approximately 21–28 nucleotide short interfering RNAs (siRNAs) by the endoribonuclease III enzyme Dicer, which is assisted by the dsRNA-binding protein TRBP. These naturally generated siRNAs have symmetric 2- or 3-nucleotide 3′ overhangs: 3′-hydroxyl and 5′-phosphate residues. In experimental as well as in potential therapeutic approaches, the endoribonucleolytic activity of Dicer is merely used for the intracellular production of siRNAs from short hairpin RNAs (shRNAs) encoded by DNA expression cassettes ( Figure 5.1 ). In a concerted reaction, siRNA is loaded into the RNA-induced silencing complex (RISC). For incorporation of siRNAs into RISC, it is necessary that siRNAs are phosphorylated at the 5′ end. RISC becomes activated by unwinding the siRNA duplex. The antisense strand of the siRNA guides RISC to the homologous target mRNA, where the endoribonucleolytic digestion by Argonaute-2 (Slicer), a nuclease residing within the RISC complex, is catalyzed. Cleavage of the target mRNA occurs at a defined site 10 nucleotides from the 5′ phosphate of the antisense strand of the unwound siRNA molecule within the center of the duplex region between the target molecule and the siRNA. Due to the loss of the 7-methylguanine cap structure of the 3′ cleavage product and the loss of the poly(A) tail of the 5′ cleavage product, the digested target mRNA is no longer protected against endogenous RNases and can be degraded.

The observation that synthetic siRNA molecules could be used to specifically silence genes in mammalian cells initiated an explosion of research on the mechanisms and application of the RNAi pathway . Following the first evidence of the in vivo efficacy of the RNAi technology in an animal model drug development efforts have been rapid, and several clinical trials, including phase III trials, with RNAi-based therapeutics are underway .
In accordance with classical RNA-based therapeutics (i.e., antisense oligonucleotides and ribozymes), the key challenge with RNAi drugs is achieving effective delivery in the tissue of interest. Thus, many of the most advanced clinical trials are designed for indications involving local delivery of siRNA molecules, for example, to the eye or the lung.
To prolong the half-life of RNA-based agents, diverse chemical modifications were developed and biochemically characterized in detail. Modifications such as alterations of the oligonucleotide backbone to protect against degradation by nucleases have proved valuable in developing therapeutic RNAi effectors. New strategies for delivery of RNAi-mediating therapeutics are continuously in development. One of these novel approaches utilizes nonpathogenic bacteria to deliver RNAi drugs to the tissue of interest. Primarily, this therapeutic concept focuses on target cells that are physiologically in contact with bacteria, for example, in the gut.
Bacteria-Mediated Cancer Therapy
The idea to utilize bacteria as an anticancer agent is not really new. In the 18th century, hundreds of cases of spontaneous tumor shrinkage following infection with bacteria were reported . As a therapeutic strategy, this concept was implemented following an intentional infection of a patient suffering on soft tissue sarcoma in 1868 with erysipelas . The result of infection with erysipelas was a rapid tumor regression, but this response was only partial and tumor recurrence occurred. At that time, bacteria were not identified as pathogen agents, and it was not known that the origin for erysipelas is an infection with microorganisms. In 1881, Streptococcus was identified as the causative agent for erysipelas. Currently, the only example of bacteria utilized for treatment of cancer is bacillus Calmette–Guérin (BCG). These attenuated bacteria are routinely used in clinics for therapy of bladder cancer .
In the 20th century, it was reported that anaerobic bacteria can target tumors and propagate within the hypoxic and necrotic regions of these malignancies . In the 1960s, it was shown that Clostridium caused tumor regression in rodent models, but a subsequent clinical trial failed to show any benefits that would compensate unwanted side effects . This strategy was improved by development of attenuated strains of Salmonella and Clostridium . In several phase I trials, a genetically modified strain of Salmonella typhimurium (VNP20009) was used to treat patients suffering from metastatic melanoma or metastatic renal cell cancer with intravenous bolus infusions of this therapeutic bacterium . The bacterium strain could be administered safely to cancer patients, and some colony formation occurred in the tumors. Disappointingly, no anticancer effects of S. typhimurium were observed. Additional studies used facultative anaerobes for tumor colonization, such as Escherichia coli and Vibrio cholera .
These primary concepts using bacteria merely utilized the natural life cycle of the microorganisms to affect tumor cells. During the same time, novel gene therapeutic concepts were developed. Accordingly, the idea of pure bacteria-based anticancer therapy was combined with the intention to design an improved therapeutic bacterial system that targets cancer cells and may be useful as a vector for transfer of therapeutic molecules, such as nucleic acids and proteins. For this approach, the well-characterized but noninvasive model organism E. coli was chosen to demonstrate its utility as a vector for transfer of nucleic acids into target cells. A diaminopimelic acid (DAP) auxotrophic E. coli strain was transformed with a plasmid encoding the genes responsible for entry, intracellular mobility, and cell-to-cell spread of Shigella flexneri . This genetically modified E. coli clone could enter mammalian epithelial cells such as carcinoma cells . In a proof-of-concept experiment, this invasive strain was used to deliver plasmid DNA into mammalian cells, including cancer cells . The bacterium-mediated DNA transfer was enabled by lysis of the invasive E. coli following entry into the host cells due to impaired cell wall synthesis that resulted from DAP auxotrophy.
This strategy using E. coli was improved by the consideration that two different steps are required for bacteria to act as delivery systems for therapeutic nucleic acids into mammalian cells: (1) internalization of the microorganisms into the host cell by endocytosis, followed by (2) escape of the therapeutic bacteria or their therapeutic nucleic acid molecules from the endocytosis vesicle to the cytosol. Thus, an E. coli strain was equipped with a plasmid containing sequences that encode two different proteins that can mediate these two steps necessary for nucleic acids delivery .
The first of these factors is the Yersinia pseudotuberculosis protein invasin. The 108-kDa protein invasin is encoded by the 3.2-kb inv locus. It mediates the entry of E. coli into the target cell. Invasin is localized on the bacterial surface and is able to bind to a subset of β 1 integrins embedded in the cell membranes of mammalian cells. This interaction enables the selective endocytotic uptake of therapeutic E. coli by the mammalian host cell. Following cellular internalization, E. coli are located in a lyosomal endocytosis vesicle or phagosome, where lysis of the microorganisms occurs.
The second protein necessary for bacterial invasion is the 50- to 80-kDa protein listeriolysin O (LLO). It is encoded by the 1.5-kb hly gene. It is a pore-forming toxin of the cholesterol-dependent cytolysin (CDC) family and a primary virulence factor of the gram-positive, facultative intracellular pathogen Listeria monocytogenes. LLO is capable of binding and perforating phagosomal membranes at low pH. The cytoplasmic contents of invasive E. coli , including therapeutic nucleic acids or proteins, can then escape into the cytosol of the mammalian host cell through the LLO-generated pores. This therapeutic strategy was successfully applied for bacteria-mediated delivery of therapeutic DNA and proteins in vitro as well as in vivo .
Delivery of RNAi Effectors by Invasive Bacteria
A major challenge for development of therapeutic RNAi effectors is the successful delivery of efficacious doses of the RNAi-triggering molecules to the target tissue. Accordingly, concepts for delivery of RNAi-based drugs were extended to the use of therapeutic bacteria strains. The principle of this strategy is the design of invasive microorganisms expressing therapeutic RNAi effectors directed against a specific disease-associated mRNA within the target cells. In this concept, an invasive bacteria strain containing a DNA vector encoding the therapeutic RNA molecules has to be designed. Although the use of expression vectors encoding single siRNA strands is possible in principle, the RNAi-mediating agents will be first and foremost shRNAs. Therapeutic shRNA molecules can be delivered by two different bacteria-using strategies. The first is transkingdom RNAi (tkRNAi) . In this concept, shRNA are delivered into target cells by invasive bacteria which themselves produce the therapeutic molecules that will subsequently become available for the target cells ( Figure 5.2A ). The second strategy is bacteria-mediated RNAi (bmRNAi) . In this approach, RNAi effectors are delivered by invasive bacteria conveying shRNA-encoding DNA constructs that will act as a matrix for transcription of the shRNA-encoding DNA sequence in the target cell by the host cell’s transcription machinery ( Figure 5.2B ).

Transkingdom RNAi
In the tkRNAi strategy (overview in Nguyen and Fruehauf ; video overview in Lage and Krühn ), nonpathogenic bacteria are applied to produce and to deliver therapeutic RNAi effectors into target cells to hijack the cellular RNAi machinery. For this approach, a specialized plasmid vector is necessary, which contains different genetic elements. First, expression of therapeutic shRNA molecules is driven by a bacteriophage T7 promoter and controlled by a T7 terminator, allowing an accumulation of the therapeutic shRNA molecules inside the bacterial cell. Second, for invasion of the target cell, the inv locus from Yersinia pseudotuberculosis is needed. The gene encodes the expression of invasin on the bacterial surface. The protein interacts with the cytoplasm membrane-embedded receptor β 1 integrin on the surface of epithelial cells. The invasin–β 1 integrin interaction triggers a rearrangement of the cytoskeleton of the target cell, resulting in the formation of a groove and an endosomal uptake of the bacteria by the cell. Third, after the bacteria enter the host cell via endocytosis, the bacterial wall is damaged by lyosomal enzymes and the therapeutic shRNAs are released from the bacteria into the vesicle lumen. In the next step, the endosomal vesicle has to be lysed to permit the therapeutic shRNA molecules to escape from entry vesicles. This membrane rupture is catalyzed by the pore-forming toxin LLO encoded by the HlyA gene from Listeria monocytogenes.
tkRNAi-designed expression vectors, such as TRIP, containing the genes for invasion and escape, inv and HlyA, as well as the therapeutic T7 promoter-driven shRNA-encoding sequence are introduced into a competent nonpathogenic E. coli strain BL21(DE3), which expresses T7 RNA polymerase. Because the tkRNAi-mediating model organism E. coli represents a physiological bug of the human gut, this tkRNAi strategy is primarily suited to treat large intestine-associated disorders such as colon cancer or chronic inflammatory bowel diseases. Thus, the first application of the tkRNAi concept was designed to target the colon cancer-associated oncogenic β-catenin-encoding mRNA . β-catenin is an integral component in the Wnt signaling pathway with oncogenic features that has been previously demonstrated to be involved in the development of the majority of colon cancers . Accordingly, the potential of β-catenin to be a colon cancer-associated pharmacological target molecule has been discussed for a long time. However, so far the use of conventional small molecule pharmacological compounds to target β-catenin has not shown satisfactory results. Thus, β-catenin appears to be an ideal target molecule for tkRNAi using the TRIP/ E. coli system. In the first proof-of-concept experiment, the human colon cancer cell line SW480 was used for in vitro exposure to anti-β-catenin shRNA-encoding TRIP-containing E. coli and then treated with different antibiotics to remove extracellular bacteria . The experiment demonstrated that β-catenin was specifically downregulated at the mRNA and protein level. Direct introduction of the therapeutic TRIP vectors into SW480 cells by transfection induced no gene silencing, further confirming that active shRNA was made by the bacteria but not by the cancer cells.
In following in vivo experiments, these observations were confirmed. The therapeutic microbes were administered to mice orally five times per week for 4 weeks. Although most of the administrated bacteria were eliminated during passage through the bactericidal environment in the upper gastrointestinal tract, a specific β-catenin downregulation could be demonstrated at the protein level by immunohistochemistry. Histological evaluation showed that β-catenin downregulation was more pronounced in the regions of, or adjacent to, the Peyer’s patches. No signs of gross or microscopic alterations with regard to epithelial damage or ulcerations were observed. Finally, the tkRNAi strategy was used through systemic injection of anti-β-catenin shRNA-encoding TRIP-containing E. coli into the tail vein of BALB/c nude mice. The treatment consisted of a total of three injections given once every 5 days. The treated nude mice carried subcutaneous colon cancer tumor xenografts derived from SW480 cells. In the xenografts, a significant reduction of β-catenin expression at the mRNA and protein level was demonstrated, with no unwanted side effects.
In another study, the potency and efficacy of the tkRNAi concept were evaluated in a completely different model system with clinical impact . The tkRNAi strategy was applied for targeting the multidrug resistance (MDR)-mediating drug extrusion pump MDR1/P-gp (ABCB1). MDR is the major cause of therapy failure of cancers with cytotoxic drugs. This phenomenon is characterized by a cross-resistant phenotype of cancer cells against several unrelated drugs that differ widely with respect to molecular structure and target specificity . The biological efficacy of the tkRNAi approach was assessed in the well-characterized human MDR gastric carcinoma cell line EPG85-257RDB. This cell model has previously been evaluated for different RNAi strategies, such as transient in vitro downregulation of MDR1/P-gp by chemically synthesized siRNA molecules, adenovirus-administered shRNAs, as well as stable knockdown by plasmid-encoded shRNAs . The shRNA sequences that were used had also shown biological efficacy for in vivo targeting of MDR1/P-gp by RNAi . EPG85-257RDB gastric cancer cells efficiently internalized therapeutic E. coli by endocytosis. The tkRNAi approach induces targeted gene silencing resulting in downregulation of the MDR1/P-gp-encoding mRNA and the corresponding cytoplasm membrane-embedded protein. Furthermore, the drug extrusion activity was inhibited and resulted in a reversion of the drug-resistant phenotype of the cancer cells. However, the extent of downregulation of the MDR1/P-gp-specific mRNA by tkRNAi was less pronounced than that observed from chemically synthesized siRNA molecules . By applying a gene therapy-like approach with plasmid- or adenovirus-encoded shRNAs, a complete knockdown of the MDR1/P-gp-encoding mRNA expression level was observed . The extent of inhibition of drug transport activity and reversal of drug resistance by the tkRNAi platform was less pronounced compared to conventional RNAi strategies, and the amount of anti-MDR1/P-gp shRNA recovered from the tkRNAi-treated gastric carcinoma cells was lower compared to that in cells treated with shRNA-encoding adenoviruses .
The tkRNAi concept was also used to design an attenuated facultative anaerobic, invasive S. typhimurium strain 7207 (SL) containing the pSLS vector encoding shRNAs directed against the β-catenin encoding mRNA . The pSLS vectors contain the HlyA gene, the T7 RNA polymerase gene, and a T7 promoter and T7 terminator-controlled shRNA expression cassette. Genetically modified S. typhimurium microbes were found to accumulate and replicate 10 3 to 10 4 times more efficiently in tumorous mouse tissue than in healthy tissues . In the experiments using tkRNAi-triggering E. coli containing the TRIP vector, the S. typhimurium -mediated tkRNAi approach resulted in a specific β-catenin downregulation in SW480 cells. Furthermore, in SW480 xenograft mice, the tkRNAi-dependent β-catenin downregulation reduced tumor growth.
In summary, tkRNAi is a powerful therapeutic strategy with the additional advantage that tkRNAi-based drugs may be orally administered. As such, CEQ508 was the first orally administered tkRNAi drug candidate filed as an investigational new drug with the U.S. Food and Drug Administration . However, in tentative experiments, the gene silencing efficacy of the tkRNAi strategy appears less pronounced compared to that of the conventional RNAi techniques. Several reasons for this observation should be considered. Probably, each of the components of the tkRNAi system leaves room for optimization, be it the bacterial biosynthesis of therapeutic shRNA molecules from the T7 promoter or the lysis of the shRNA containing entry vesicles and the possible damage to the shRNA through chemical modification in the entry vesicles. Variable lengths of the shRNA could lead to inefficient Dicer processing and influence the amount of resulting active siRNAs. Each of these factors may have contributed to a lower gene silencing efficacy and might present an opportunity to build improved versions.
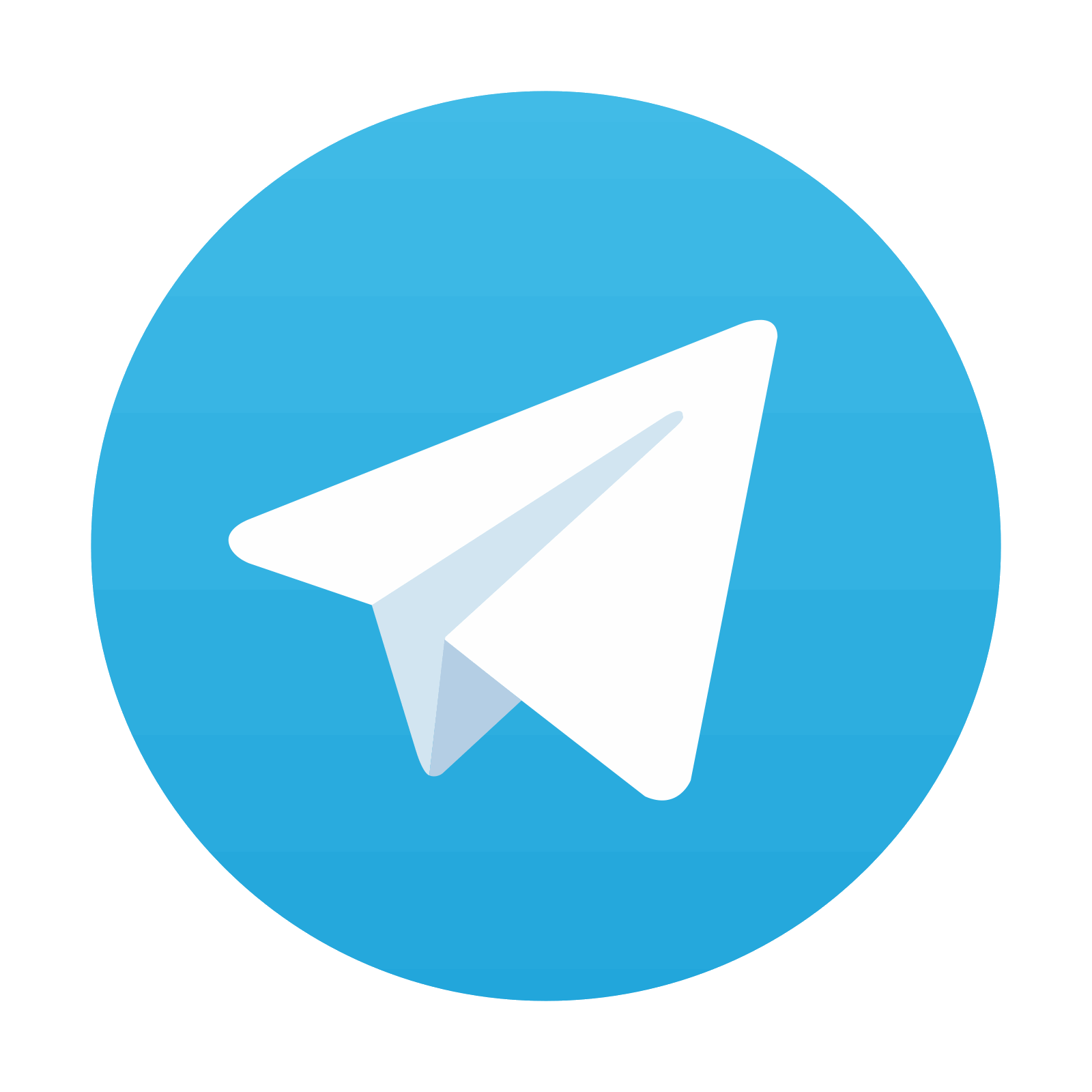
Stay updated, free articles. Join our Telegram channel
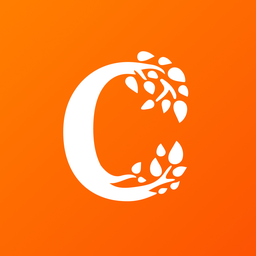
Full access? Get Clinical Tree
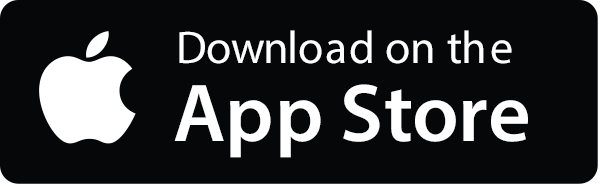
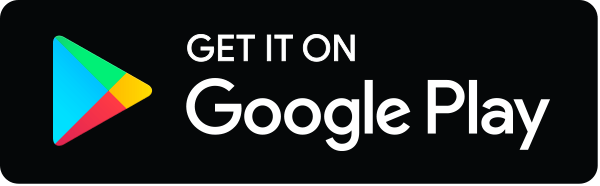