Method
Measurement
Advantages
Disadvantages
Value in drugdevelopment decisions
DXA
Density of whole body tissue to determine the separate composition of fat tissue, lean soft tissue, and bone mineral content/density
Short scan time (5–10 min); open table configuration; user-friendly software; easy to standardize
X-ray exposure; table weight limit (~300 lb; ~136 kg); table scan limit (~60 cm width); cannot separate different types of adipose tissues; technologists may be unfamiliar as this is not usual standard of care
Suggested by FDA (Food and Drug Administration) in Draft Guidance as general safety assessment for reduction in fat content not lean-body mass
CT
Density of various tissues in order to produce anatomical images for the assessment of normal and abnormal body structures
Cross-sectional imaging; larger bore size (70–90 cm); higher table weight limit (~400–450 lb; ~182–2,014 kg)
X-ray exposure; technologists typically rotate between modalities; more difficult to standardize
Should be used for direct VAT* measurement, rather than waist circumference, as a surrogate measure
MRI
Water content of various tissues in order to produce anatomical and dynamic images for the assessment of normal and abnormal body structures and function
Cross-sectional imaging; no X-ray exposure; higher table weight limit (~350 lb; ~159 kg); technologists are more consistent
Smaller bore sizes (60–70 cm); claustrophobia risk; smaller field of view (~50 cm); longer scan times (~30 min); more difficult to standardize
Should be used for direct VAT measurement, rather than waist circumference, as a surrogate measure
Image Analysis
Method | Measurement | Advantages | Disadvantages | Value in drug development decisions |
---|---|---|---|---|
DXA | Separation of anatomical regions of interest to quantify fat tissue, lean soft tissue, and bone mineral content/density | Auto-analysis features available; manual analysis procedures are software driven; easy to develop a protocol | Site technologists are not typically familiar with body composition scan, as they are not standard of care; sites often perform local image analysis according to their established procedures and independent of other sites | FDA Draft Guidance suggests centralized process can better provide verifiable and uniform training, management, and performance |
CT | Quantification of internal organs or tissue volumes, as well as some qualitative measurements | Thresholding by Hounsfield units; some automated segmentation available; can be performed on existing PACS/workstations | Analysis by CT technologists is inefficient and often interrupted by scanning responsibilities; clinical software is typically for routine measurements, not rigorous analysis | FDA Draft Guidance suggests centralized process can better provide verifiable and uniform training, management, and performance |
MRI | Quantification of internal organ or tissue volumes, as well as a range of dynamic, functional, and qualitative measurements | Wider range of acquisition protocols allow for a larger number of quantitative and qualitative analyses | Images are more varied and complicated to analyse; fewer options for automated analysis; strict protocols must be followed in order to reduce individual analyst bias | FDA Draft Guidance suggests centralized process can better provide verifiable and uniform training, management, and performance |
Conclusions
Body composition imaging is more technical than general clinical imaging and should be constrained to a rigorous protocol in order to provide reproducibility and scalability for translational research. Since image acquisition and processing technologies are rapidly evolving, researchers and scientists should thoroughly evaluate logistical concerns and scientific needs before selecting an imaging modality. As with any scientific methodology, the quality and validity of results are only as good as the source data; therefore, engineers, physicists, and technologists should be included in discussions with clinical researchers in order to address technical issues that must be resolved for use in translational research. Body composition imaging has long been utilized for obesity/diabetes research and drug development and is expanding into other disease and therapeutic areas. Broader implementation will increase the familiarity and quality of these techniques while building a solid foundation for new investigations.
Introduction
The Pathophysiology of Obesity or Diabetes
Adipose tissue mass and function are closely associated with health-related conditions such as impaired insulin sensitivity, metabolic syndrome [1], and type 2 diabetes [2]. The utilization of medical imaging has allowed for a better understanding of the effects of obesity and diabetes and has also increased our understanding of the mechanism of these conditions. Hyperglycaemia, impaired postprandial insulin secretion, elevated fasting plasma insulin levels, increased fatty acids and triglycerides, and insulin resistance are associated with adiposity but more specifically, with certain patterns of adipose tissue and fat distribution.
The measurement of visceral adipose tissue (VAT) by computed tomography (CT) and magnetic resonance imaging (MRI) has allowed for the breakdown of abdominal adiposity into separate VAT and subcutaneous adipose tissue (SAT) compartments, but also for further subdivision into more detailed and distinct anatomical depots [3]. As a single depot, VAT is strongly associated with insulin resistance of skeletal muscle, as well as with dyslipidaemia, and increased risks for hypertension and glucose intolerance [4]. Differences in the amount of VAT have been determined to account for a significant amount of the variability in the severity of insulin resistance and also have a stronger correlation than fat mass [5] (Fig. 6.1).
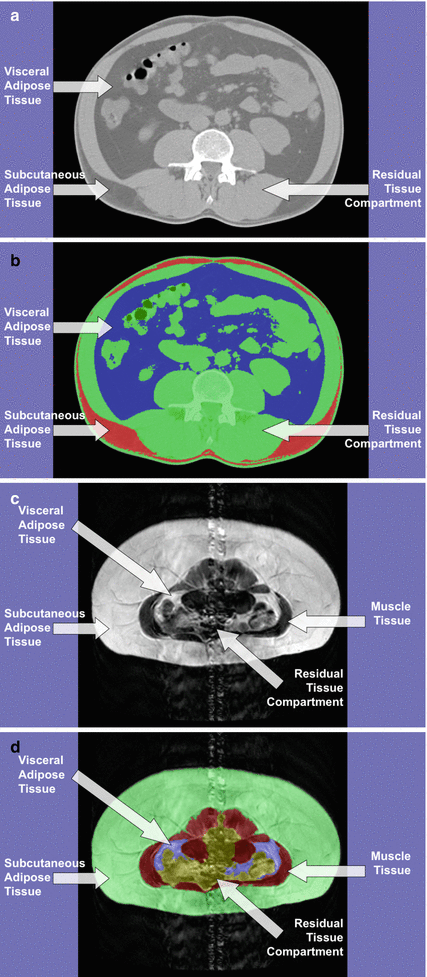
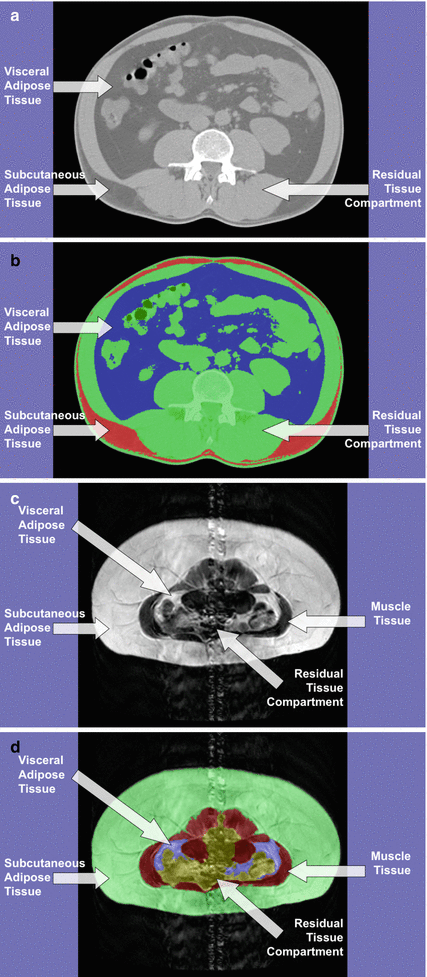
Fig. 6.1
(a) CT image acquisition. (b) CT Analysis for SAT and VAT. (c) MRI image acquisition. (d) MRI analysis for muscle, SAT, and VAT
The high resolution of cross-sectional imaging has allowed for the identification of an established fascial plane that separates SAT into deep and superficial layers, which are known to have distinct histological properties. The deep SAT layer has been shown to have an association with insulin resistance that is similar to VAT, which the superficial SAT layer has a weaker association [6] (Fig. 6.2).
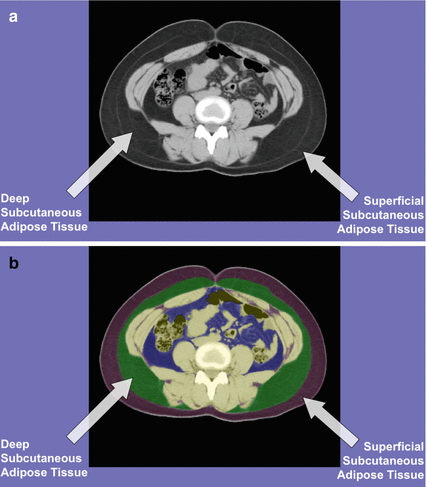
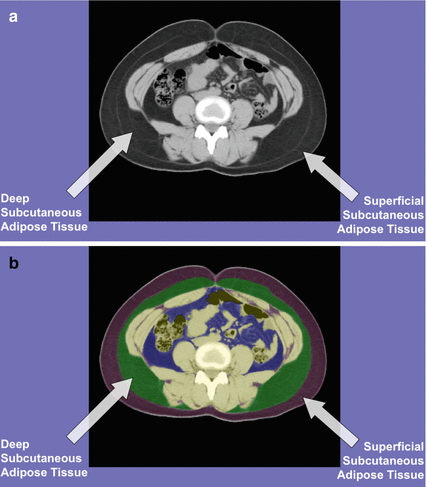
Fig. 6.2
(a) CT image of abdomen. (b) CT analysis for deep and superficial SAT
Intermuscular adipose tissue (IMAT) has also been identified as adipose tissue located beneath the muscle fascia and combined with contiguous depots of adipose tissue between muscle bundles [7]. IMAT has been show to be significantly correlated with insulin resistance in middle-age [8] and older adults [1, 9]. Continued interest is focused on the qualitative analysis of other tissue or organ composition, such as skeletal muscle and liver, where higher fat content has been identified in obesity and type 2 diabetes (Fig. 6.3).
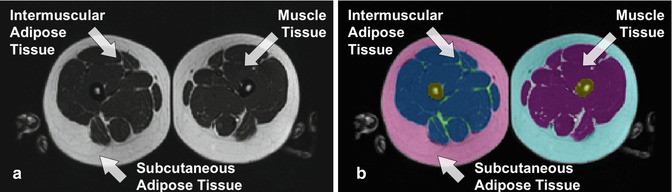
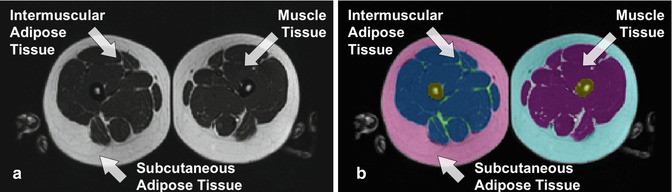
Fig. 6.3
(a) CT image of thigh. (b) CT analysis for muscle, IMAT, and SAT
The Development of Pharmacological Agents
Drug distribution within the body is dependent on a number of factors including blood flow, body composition, fluid distribution, and protein/tissue binding. It is possible for drug distribution to shift as protein concentration and body composition changes occur. Once in the blood, circulation throughout the body can be affected by regional flow in the central compartment of high metabolic rate organs (brain, heart, kidney, and liver) and the peripheral compartment of less well-perfused organs (adipose and muscle tissue). For example, despite the fact that anaesthesia is metabolized slowly, the duration of effect is shortened because drug distribution into adipose tissue acts as a storage site or drug reservoir.
Since phenotypic and lifestyle changes of body composition and mass can affect drug distribution and metabolism, the use of imaging modalities to assess the variability and magnitude of the changes early on in the drug development process allows for more efficient clinical trials and more valuable clinical results. The Food and Drug Administration (FDA) has included the use of imaging biomarkers as endpoints for quantifying the size of effect in its Guidance for Industry Standards for Clinical Trial Imaging Endpoints. More specifically, the Guidance for Industry Developing Products for Weight Management incorporates the use of DXA, CT, or MRI for body composition assessment:
To ensure that drug or biologic-induced weight loss is caused primarily by a reduction in fat content, not lean-body mass, a representative sample of study subjects should have a baseline and follow-up measurement of body composition by DEXA, or a suitable alternative.
Because the evaluation of investigational weight-management products routinely includes assessment of changes in patients’ metabolic profiles, and in some cases may involve measurement of visceral fat content by CT or MRI, waist circumference should not serve as a surrogate for visceral fat content when measured in a clinical trial investigating the efficacy of a product for weight loss.
An additional area of focus for drug development includes the imaging modalities of positron emission tomography (PET), functional MRI (fMRI), and magnetic resonance spectroscopy (MRS) for investigations of mechanisms and in vivo metabolism. These techniques allow for the assessment of tissue and organ quality and composition, fuel utilization, oxygen uptake, blood flow, and other functional properties.
Background
The assessment of body composition for research began in the late 1800s with basic investigations of the human body for fat, protein, salt, and water content [2, 10, 11], while the early 1900s moved into more specific quantifications on the atomic, chemical, and molecular level for the whole body [3, 12–14]. In 1942, Behnke et al. reported on the estimation of lean vs. fat by Archimedes’ principle and introduced the two-compartment model and underwater weighing method, which could be implemented by other investigators [4, 15]. With advances in science and technology, the 1960s and 1970s saw development of the three-compartment model by Siri [5, 16] and the introduction of new methods and devices, such as bioimpedance analysis and dual-photon absorptiometry [6, 17, 18]. In 1979, Heymsfield et al. were the first to utilize CT for body composition imaging [7, 19].
Increased clinical adoption of medical imaging in the 1980s allowed body composition assessment to expand from single and multiple slices to whole body imaging, as well as from CT to MRI [8, 20–23]. In the 1990s, continued refinement and cross-validation of the different research methodologies led to the commercial development of bioimpedance analysis (BIA), air displacement plethysmography (BOD POD), and dual-energy X-ray absorptiometry (DXA) systems with widespread clinical, consumer, and research utilization. With the current variety of research techniques available for the assessment of body composition, logistical and scientific considerations play an important role in the selection process. At one end of the spectrum, anthropometric measurements are commonly utilized as surrogates of total body adiposity, where skinfold thickness is a low-cost field measurement, but has a considerable amount of variability in the subcutaneous thickness and compressibility of adipose tissue for a given measurement location. Intermediate techniques, such as the BODPOD or DXA, are more accurate and advanced than the field measures, but still only distinguish between two (fat and fat-free mass) and three (bone, fat, and lean mass) components of body composition, respectively. At the sophisticated end of the spectrum, imaging modalities such as CT and MRI are able to directly quantify adipose tissue in specified regions or the whole body with higher accuracy, but also higher costs and technical requirements.
Key Methods
Body composition imaging has been utilized for over 35 years in scientific research, but has yet to be implemented as a routine clinical measurement. Frequently designated as “non-standard of care”, body composition imaging can be classified as a highly technical imaging technique. Since the average imaging technologist does not frequently perform these types of specialized scans in routine clinical practice, there is a need for comprehensive training where emphasis is placed on a number of factors including adherence to a specified acquisition protocol, maximum reproducibility and minimum variability between timepoints, and strict accountability for documentation and transfer of source data. The most accurate and precise use of these imaging techniques in translational research requires an evaluation of the type and maintenance of equipment to be utilized, the experience and training of imaging technologists who will perform the scan, and the protocol to be utilized for acquiring the images and managing the data.
The key methods are organized by imaging modality and typically selected based on the prioritization of logistical concerns versus scientific need. The less complicated methods will be more readily available and can be implemented in a more scalable manner, but only provide a limited number of results. The more advanced techniques require higher end scanners and additional equipment, which are most often located at academic medical centres or large imaging facilities, but are able to produce additional results which are not directly available from other modalities.
Dual-Energy X-Ray Absorptiometry
Methods
DXA is widely accepted for its ability to measure bone mineral density and utilized for the assessment of fracture risk and the diagnosis of osteoporosis from lumbar spine, proximal femur/hip, and distal forearm scans. Broad adoption has lead to the installation of approximately 50,000 whole body DXA scanners worldwide and, because of increased accessibility, the utilization in numerous studies ranging from bone health, exercise and HIV/AIDS to diabetes, muscle wasting/sarcopaenia, and obesity/weight loss. The majority of DXA scanners also include the whole body scan mode for body composition, which emits a low radiation dose between 0.02 and 1.5 mrem, depending on the instrument and the scan speed, and is less exposure than acquired during one transcontinental flight across the USA (4–6 mrems). Since some radiation is involved with all DXA scans, it is contraindicated for women who might be pregnant and requires administration of a pregnancy test prior to scanning in women of childbearing age.
A whole body DXA scan can measure three main body composition compartments (bone mineral content, fat mass, and lean soft tissue mass), divided into separate anatomical sections for arms, legs, and trunk. The typical acquisition time is approximately 15 min for a whole body scan, which is comprised of 5 min for entry of patient biography, 5 min for patient positioning in supine orientation, and 5 min for image acquisition. Patients should be properly screened to ensure that they would be able to fit within the height, weight, and width limitations of the DXA scanner. The weight limit is typically 300 lb (~136 kg) for most DXA scanners, but newer generations have improved table designs to accommodate up to 450 lb (~205 kg). Patients taller than 76 in. (193 cm) or wider than 23–26 in. (58–65 cm) might not fit within the imaging field of view, and some tissue or an entire limb region could be rendered invalid data. Therefore, careful positioning is crucial for the acquisition of reproducible scans to ensure the accuracy and precision of the resulting image analysis.
Standardization of patient preparation should include proper control of hydration status by conducting serial measurements at the same time of day, under fasting conditions, and during the same period of the menstrual cycle for females and wearing a hospital gown after removing jewellery and any undergarments that might contain metal clasps or wires. Positioning should be standardized by ensuring:
1.
Patient is resting comfortably in the supine position.
2.
Body is centred evenly on the table pad.
3.
There is approximately 1–2 in. of space between the top of the head and the topline of the table pad.
4.
Hands are placed in a lateral position beside the body.
5.
Legs are straight with feet perpendicular to table and toes together.
6.
All body parts and tissue are within the field of view (Fig. 6.4).
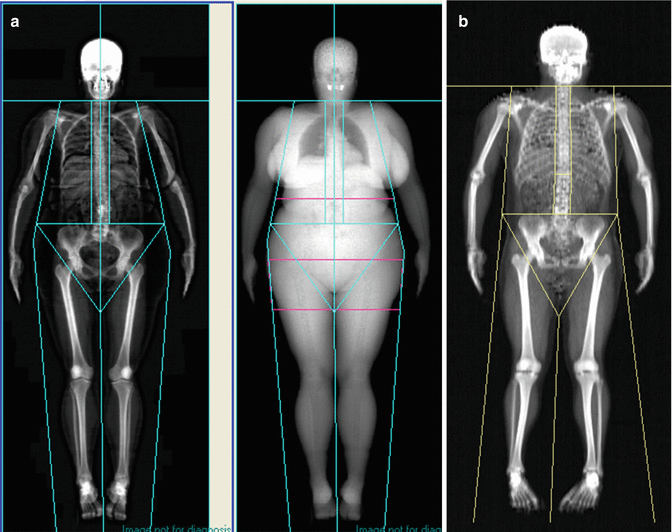
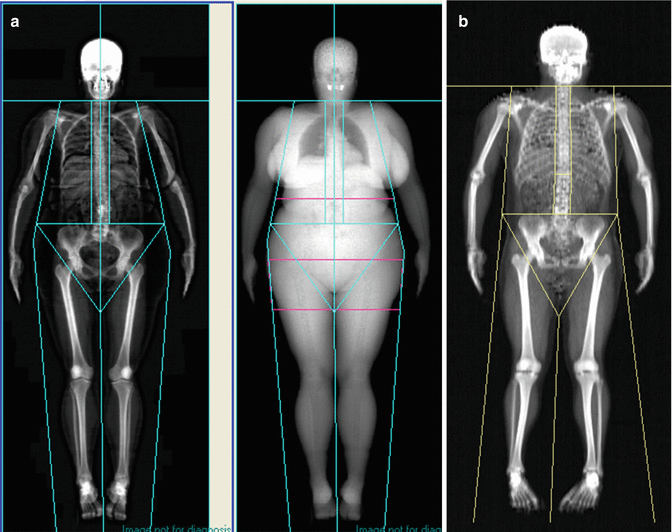
Fig. 6.4
(a) GE lunar whole body DXA. (b) Hologic whole body DXA
Some accommodations can be made to wrap a patient’s chest, abdomen, and hip regions with a thin sheet in an attempt to keep the tissue within the field or view or to place a small folded pillowcase between arms and hips or between thighs in order to keep separate tissue from overlapping. If a patient is too large to fit within the field of view, even after multiple attempts to reposition and wrap, then scans should be acquired on the right half of the body so that total body composition can be estimated, based on the principle of bilateral symmetry [24] (Fig. 6.5).
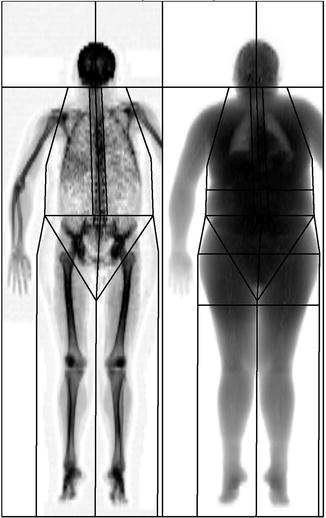
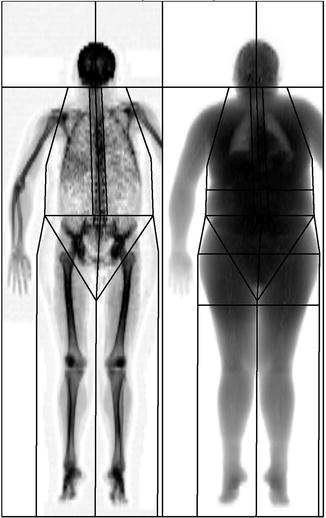
Fig. 6.5
DXA hemi-scan
For a valid, completed DXA scan, it is an important quality control (QC) step to compare the measured scale weight to the sum of all DXA subregions. The average agreement between measurements should be within a 2.2 lb (1 kg) standard deviation [25]. Additional calibration and quality assurance (QA) procedures utilize different phantoms and standards depending on the scanner manufacturer, model, and software version. Different forms of calcium hydroxyapatite and aluminium spine phantoms are either included by the scanner manufacturers or available from third parties (“Bona Fide Phantom” from BioClinica or “European Spine Phantom” from QRM GmbH) for daily QC as well as monitoring long-term scanner stability. Phantoms with components to mimic soft tissue include the Hologic “Whole Body Phantom”, the BioClinica “Variable Composition Phantom”, and the OrthoMetrics “Whole Body Phantom” and are used for body composition measurements and cross-calibrations between similar systems (Fig. 6.6).
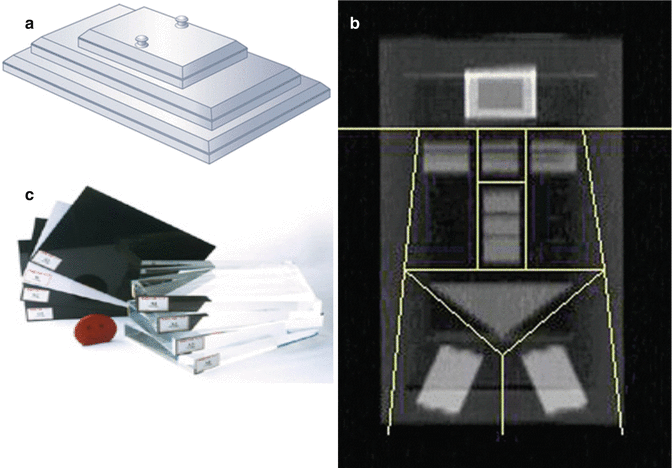
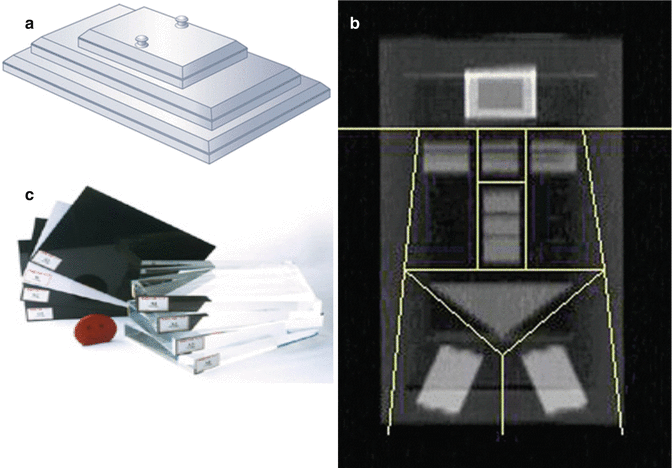
Fig. 6.6
(a) DXA whole body phantom. (b) DXA whole body phantom image. (c) DXA variable composition phantom
While most US states require a licensed radiology technologist to acquire DXA scans, this does not mean they will have experience with clinical trials and understand the importance placed on accuracy, precision, and reproducibility. Therefore, comprehensive training and standard operating procedures for the DXA technologist are essential for the proper instruction and conduct of data management activities, positioning of subjects, and scan acquisition. Additional recommendations for consistent scanning include:
1.
Serial measurements should be acquired on the same DXA scanner.
2.
All baseline and follow-up timepoints should be acquired by the same DXA technologist and utilizing the same scans mode and settings.
3.
Patient positioning should follow a standard operating procedure (SOP) from the scanner manufacturer or a study protocol.
4.
Image analysis should follow an SOP for the consistent placement of the regions of interest (ROIs) and the “compare” or “copy” function should always be used when available
5.
Auto-analysis features should also be used, but still checked by the DXA technologist, to ensure proper positioning and catch any errors
Advantages
As a relatively inexpensive imaging modality, compared to CT or MRI, DXA scanners are available for between US $75,000–$100,000, with an annual service contract around US $10,000. Widespread clinical use for osteoporosis means that a large install base of scanners is available for research-focused measurements of body composition. An open table design, minimal patient preparation, and quick scan time all contribute to a user-friendly experience for the patient. A highly automated and guided workflow with a limited number of options/settings creates a user-friendly experience for the technologist. DXA has long been accepted as a precise and reliable measurement of body composition when serial examinations are performed on the same scanner [26]. Reliability and repeatability data from our centre include coefficients of variation (CVs) for total body tissue mass (0.6 %), fat mass (2.1 %), lean mass (1.15 %), and bone mineral content (1.1 %).
Disadvantages
Inherent to DXA is the use of dual-energy X-rays, which exposes the patient to radiation. Although the dose is very low, DXA is contraindicated for women who might be pregnant. While licensed radiology technologists are often required to perform a DXA scan, they are typically responsible for multiple/other imaging modalities and clinical protocols (i.e. bone density scans for osteoporosis), but not always experienced in body composition assessment and clinical trials. As DXA is a mature imaging modality, some legacy systems are often stand-alone computer and scanner systems and not connected to a network. DXA technologists are not always up-to-date on the latest technology developments and quickly become unfamiliar with newer methods to archive and transfer data/scans from their systems.
Although the market is dominated by two main scanner manufacturers, GE Lunar (GE Healthcare, Madison, WI, USA) and Hologic (Hologic, Inc., Bedford, MA, USA), there is still a lack of standardization between the two, as well as a difference in bone mineral content of approximately 20 %. Cross-calibration equations have recently been developed and validated in over 200 individuals ranging in age from 6 to 81 years to allow for the conversion of measurements between specific software versions from the manufacturers [27].
Finally, DXA is only capable of measuring total fat within an individual pixel or specified regions and is therefore unable to differentiate between layers of adipose tissue (intramuscular, subcutaneous, and visceral) occupying the same region. Additionally, in regions with a significant amount of bone surrounding soft tissue (i.e., head, chest, pelvis), DXA is unable to separate the soft tissue into fat or lean components. Because of these limitations, cross-sectional imaging modalities, such as CT and MRI, are utilized for further tissue discrimination.
Value in Drug Development
DXA is an imaging modality that is precise and reliable for body composition measurement when serial examinations are performed on the same scanner. It is more objective than manual field methods such as body mass index (BMI), skinfolds, and waist-to-hip ratio and also provides more scientific information with regards to tissue composition and anatomical regions. DXA scanners are widely available and can be incorporated into clinical trials for drug development in a very standardized manner.
Advances in the development of new subregions for the whole body scan have been incorporated in the latest software revisions from both GE and Hologic and include discrimination of the android and gynoid fat regions, as well as the estimation of VAT. Recent publications have reported on the relationship of the android and gynoid regions to metabolic risk factors (hypertension, impaired glucose tolerance, and elevated triglycerides) [28, 29]. Both manufacturers have also developed an estimation of VAT by cross-validation against CT measurement of VAT area for Hologic [30] and VAT volume for GE [31], which demonstrate high correlations and should show increased utilization due to the number of scanners already in place (Fig. 6.7).
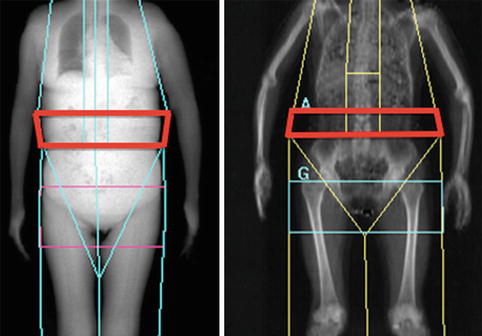
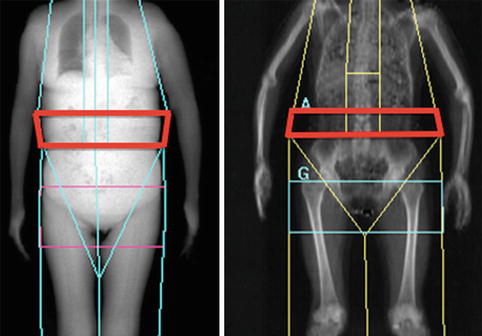
Fig. 6.7
DXA-VAT region (Left, GE; Right, Hologic)
Cross-Sectional Imaging
While field measures and intermediate imaging techniques like DXA and ultrasound (US) are widely available, their usefulness becomes limited to correlations and estimates once the research focus turns to the internal components of body composition. In order to perform direct quantitative and qualitative measurements of internal organs (brain, heart, liver, kidneys, spleen, pancreas) and tissues (muscle, SAT, VAT, intermuscular adipose tissue (IMAT)), an advanced imaging modality such as CT or MRI should be utilized [32, 33]. Typically, two-dimensional images are acquired and analysed for area in the axial, coronal, or sagittal planes and then integrated along with their slice thickness and any gaps or spacing to reconstruct a three-dimensional volume. These methods are the gold standard techniques for the measurement of adipose tissue compartments and offer new insights into associations between intra-abdominal adipose tissue and metabolic factors.
Computed Tomography
Methods
CT scanners are widely available (~35,000 worldwide) due to a large install base at hospitals and imaging facilities, as well as utilization for clinical diagnostic imaging. Even though CT does expose individuals to ionizing radiation, it is one of the only available methods (along with MRI) to be able to separate individual organs from VAT, VAT from SAT, as well as muscle from IMAT [3, 34]. The classical CT scan for body composition (SAT/VAT) is a single, axial slice acquired at the anatomic level of the L4–L5 intervertebral space, while for muscle and IMAT it is a single, axial slice acquired at the level of the midthigh, which needs to be determined by measurement and calculation from anatomical landmarks (distance between the anterior superior iliac crest and the inferior margin of the patella) [8, 35]. When these types of scans are acquired with standard settings (120 kVp, 200 mA, and 2 s exposure), the typical radiation dose ranges between 40 and 400 mrem, depending on the total amount of body volume scanned and the spatial resolution required. In comparison, CT scan can be approximately 250 times more radiative than DXA, but provides information and results not available from DXA. Since radiation is involved with all CT scans, it is contraindicated for women who might be pregnant and requires administration of a pregnancy test prior to scanning in women of childbearing age (Fig. 6.8).
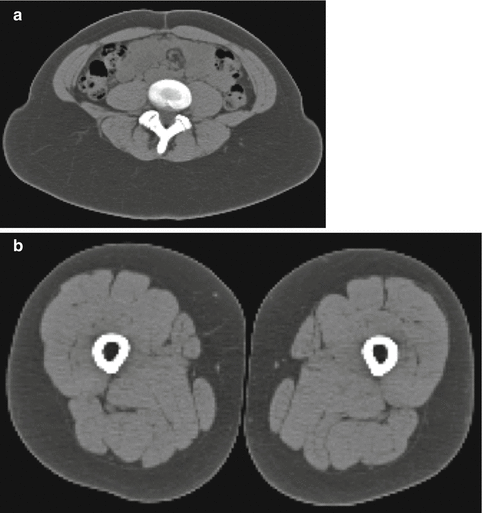
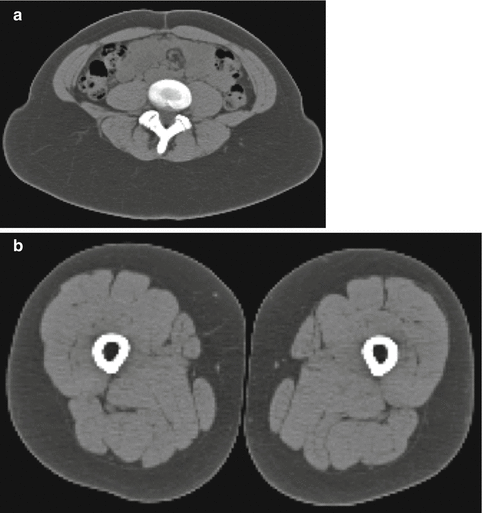
Fig. 6.8
(a) Abdominal CT image. (b) Midthigh CT image
A combined abdomen and midthigh CT scan can typically be acquired within a 30 min appointment slot, comprised of 5 min for entry of patient biography, 5 min for patient positioning in supine orientation, 5 min for acquisition of an abdominal localizer image and a single or multiple axial images in the abdomen, 5 min for acquisition of a thigh localizer image, and then 10 min to accurately measure, calculate, and acquire a single or multiple axial images of the midthigh. When acquiring a thigh scan or midthigh slice, approximately 10–15 min should be allocated for the patient to rest in the supine position and ensure an even fluid distribution for the tissue of interest.
Patients should be properly screened to ensure that they would be able to fit within the height, weight, and width limitations of the CT scanner. The typical weight limit is approximately 400–450 lb (~182–204 kg) depending on the make and model of CT scanner, but newer generations with bariatric options or larger bores have improved table designs to accommodate up to 500–650 lb (~226–294 kg). Patients wider than 21–26 in. (55–65 cm) might not fit within the imaging field of view, and some tissues might not be quantified. Therefore, careful positioning is crucial for the acquisition of reproducible scans to ensure the accuracy and precision of the resulting image analysis. Standardization of patient preparation should include wearing a hospital gown after removing jewellery and any undergarments that might contain metal clasps or wires.
While there are no specific CT QC procedures for body composition, each scanner should employ a standard phantom for daily QA activities and follow American College of Radiology (ACR) guidelines or maintain ACR certification. These standard phantom scans will allow for the longitudinal monitoring of scanner performance during the course of a study. Anthropomorphic phantoms are available, with accurate anatomical structures, where synthetic organs and tissues have radiation absorption properties approximate to human tissue and allow for scanning under actual clinical conditions. These advanced phantoms can be used for cross-calibrations between scanners (Fig. 6.9).
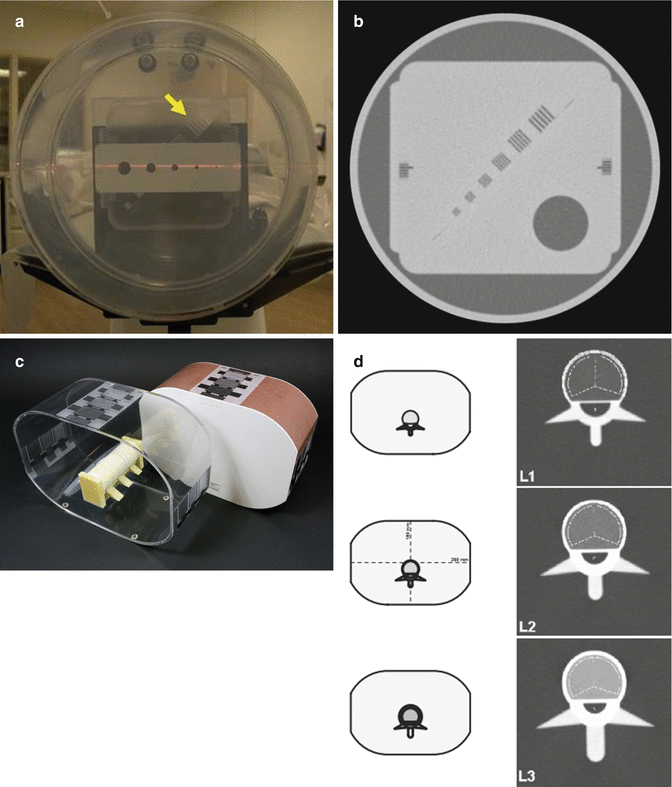
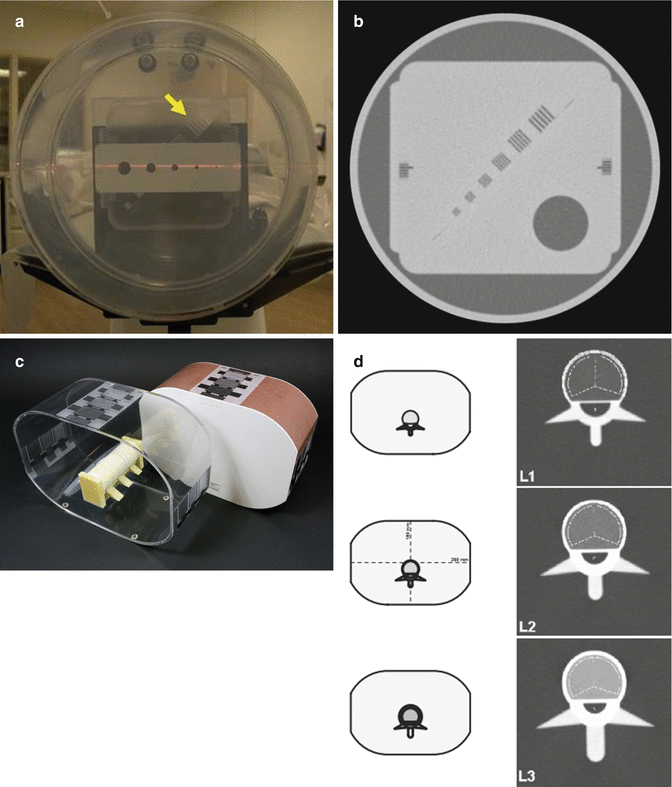
Fig. 6.9
(a) CT phantom. (b) CT phantom image. (c) EU spine phantom from QRM. (d) EU spine phantom image (Images reprinted with permission from ORM GmbH, Moehrendorf, Germany)
Additionally, a standard CT scanner can acquire scans for bone density measurements, quantitative computed tomography (QCT), by utilizing a special calibration phantom (which is scanned with the patient and contains five standards of varying density to be compared against the bone of interest), a quality control phantom to monitor system stability, and separate analysis software (Fig. 6.10).
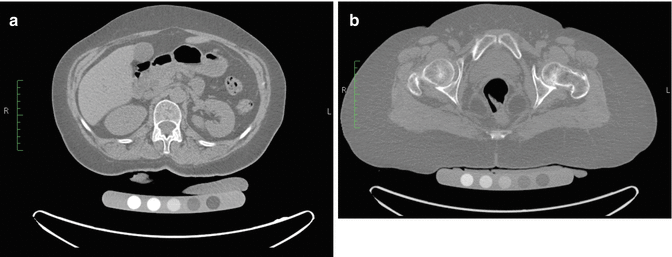
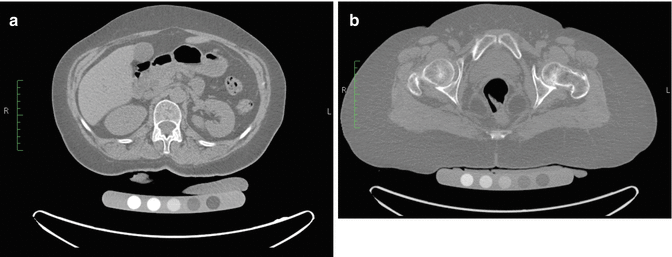
Fig. 6.10
(a) QCT spine scan. (b) QCT hip scan
A licensed radiology technologist is required to operate a CT scanner, but the technologist is often assigned to different locations and/or different scanners throughout an imaging facility, depending on staffing needs. The rapid imaging and throughput capabilities that make CT popular have also lead to shorter appointment times (sometimes as little at 15 min) and less opportunity for the technologist to focus on study documentation. As a result of these time limitations, the CT technologist will have very little flexibility for research scans, and certain activities should be completed either proactively, prior to the scan (case report forms, data transmittal forms, demographic documentation, etc.), or immediately after completion (image data transfer, image analysis).
Since a CT scanner is most often utilized for clinical scans, it is rare that a technologist will have experience with clinical trials; therefore, comprehensive training and standard operating procedures which focus on accuracy, precision, and reproducibility are essential for the proper instruction and conduct of research studies. Additional recommendations for consistent scanning include:
1.
Serial measurements should be acquired on the same CT scanner.
2.
All baseline and follow-up timepoints should be acquired by the same CT technologist and utilizing the same scan mode and settings.
3.
Images from previous timepoints should be reviewed to help maximize reproducibility.
4.
Patient positioning should follow a standard operating procedure (SOP) from the scanner manufacturer or a study protocol.
Advantages
A CT scanner is more expensive than field measurements and intermediate imaging methods (DXA and US), but allows more advanced measurements of internal organs and tissues, as well as the separation between similar tissues (IMAT, SAT, and VAT). Compared to MRI, a CT scanner is middle tier based on cost of US $100,000–$300,000, with an annual service contract ranging between US $50,000 and 100,000. CT scanners have a wide bore and short length, which accommodates larger patients while reducing the concern for claustrophobia. Individual images can be acquired in seconds and complete scans can be acquired within 5 min or less. This rapid scanning speed improves image quality, decreases breatholding, and reduces patient discomfort and time. Additionally, patients who have metal in their body (certain types of implants, metallic fragments, cardiac monitors or pacemakers) can often be scanned by CT when they would otherwise not be able to be scanned by MRI due to the magnetic field.
Another advantage of CT imaging is the objectivity of the technique, where physical density is the main determinant of X-ray attenuation, called the Hounsfield unit (HU), and each pixel is assigned an HU value on a grey scale that reflects the composition of the tissue (where air = −1,000 and water = 0). The spatial resolution and detail of a CT image allows for separation of adipose from muscle tissue, different regions of adipose tissue, as well as regions within subcutaneous adipose tissue (deep vs. superficial SAT). Simple image analysis can be performed directly on the CT scanner, on a picture archiving and communications system (PACS), or on a personal computing (PC) workstations with commercial off-the-shelf software, by utilizing a histogram or thresholding tool to select pixels within a given HU range (−190 to −30 HU for AT, 0–100 HU for muscle) and then multiplying the area of each pixel by the number of pixels [36].
Disadvantages
Costing approximately US $1,000–2,000, a CT scan is more expensive than a DXA scan (approximately US $300) and several field measures (Bioelectrical Impedance Analysis/Skin Folds) combined. Radiation exposure is a disadvantage for the use of CT, but also becomes increased in obese patients for deeper tissue penetration in order to obtain good image quality. CT scanner table weights are higher than for DXA, but still a limitation for obese patients. A limited field of view up to 70 cm for some new CT scanners can still result in tissue being excluded from imaging and analysis.
CT scanners are primarily utilized for clinical medicine and less frequently available for clinical research. Typically high daily caseloads, due to short scan times, lead to low appoint availability and scheduling difficulties for research cases, which can be intensive and require extra time to complete. CT technologists are more focused on clinical cases, where they utilize their individual knowledge and experience to obtain the best possible diagnostic image from their perspective, which is counter to the reproducible and standardized procedures required for clinical research. Technologists and managers are rarely provided enough time to learn new research techniques that are beyond standard of care imaging, to complete the required study documentation, or to manage data transfer of scans via systems outside of their PACS.
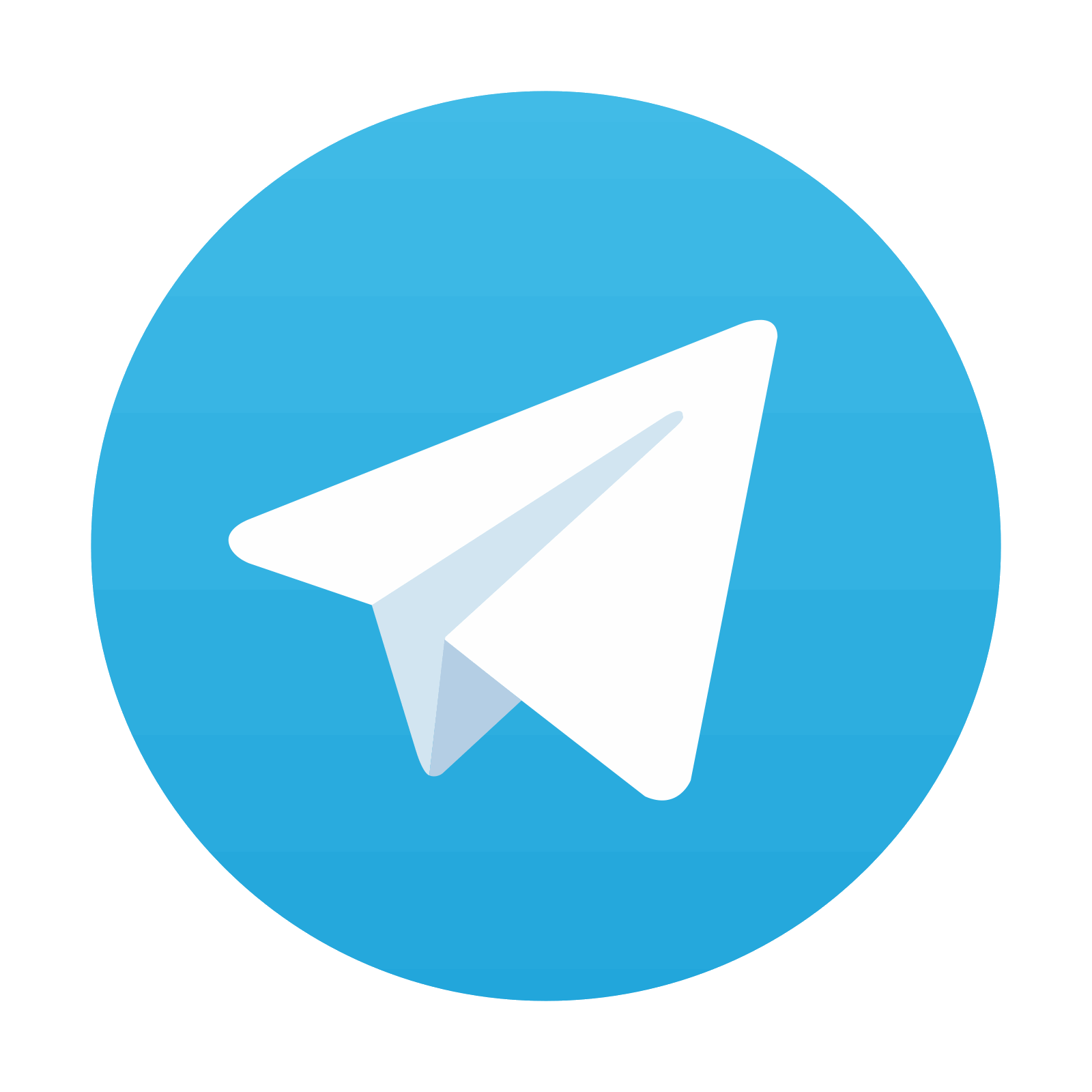
Stay updated, free articles. Join our Telegram channel
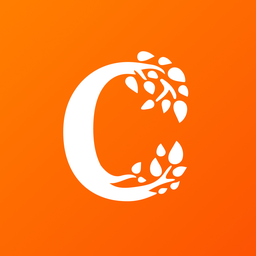
Full access? Get Clinical Tree
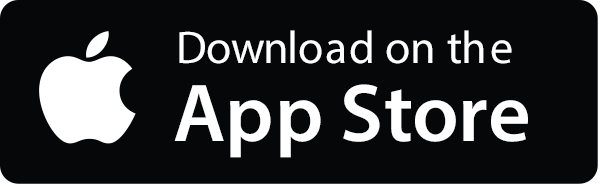
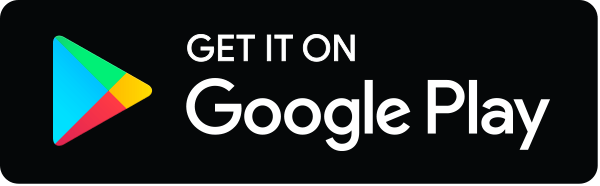