Wild-type
Aneuploid strains
Method
Notes
Haploid (1n)16 chr
Monosomies (n − 1)
Centromere silencing (CS)
Inviable
Disomies (n + 1)
CS, Chromosome transfer (CT) and meiois of 3n or 5n (RM)
12 disomes from CT characterized in depth
Multiple disomies (1n + x), (x = 2 − 6)
CT and RM
5 strains from RM characterized in depth
Haploid (n + YAC)
CT
Yeast artificial chromosome (YAC) with mammalian DNA
Diploid (2n)32 chr
Monosomies (2n − 1)
CS
Unstable, endoduplication
Trisomies (2n + 1)
CS, CT and RM
Multiple trisomy (2n + x), (x = 2 − 8)
RM
One approach utilizes chromosome transfer followed by selection to generate haploid yeast cells harboring an extra copy of a single chromosome (henceforth disomes) (Conde and Fink 1976; Hartwell et al. 1982). Indeed, a total of 13 out the possible 16 disomes were generated using this strategy (Torres et al. 2007). Because aneuploidy increases chromosomal instability (discussed below), aneuploid yeast strains generated by this method are maintained under selection. Interestingly, four additional karyotypic stable strains were obtained that carry one or two extra chromosomes in addition to the one that was selected for. To circumvent potential artifacts of selection in studying the effects of aneuploidy on cellular physiology, disomes can be grown in non-selective media for a small number of generations while maintaining their original karyotype. A second approach to generate aneuploid yeasts is through meiosis of triploid (3n) or pentaploid (5n) strains. Segregation of homologous chromosomes during meioisis I of these polyploid strains happens randomly, thus generating various aneuploidies. Aneuploid strains recovered from this method show reduced viability (15–50 %) and high genomic instability leading to heterogeneous populations (Parry and Cox 1970; Pavelka et al. 2010; St Charles et al. 2010; Zhu et al. 2012). Nonetheless, a total of 38 semi-stable aneuploid strains have thus far been recovered and their phenotypic properties analyzed (Pavelka et al. 2010). Of these, five strains were characterized in depth. Using a third approach missegregation of a single chromosome can be induced by centromere silencing (Anders et al. 2009; Reid et al. 2008). In haploid yeast, this method generates unviable monosomies and disomic strains. The latter strains were utilized to map genetic traits to specific chromosomes but other phenotypes were not analyzed. In diploids, centromere silencing of one of the homologous chromosomes generates trisomies (2n + 1) and monosomies (2n − 1). Of note, monosomies recovered from this method were shown to be unstable as colonies from these strains were heterogeneous in size and endoduplication of the entire missing chromosome was frequently observed (Reid et al. 2008). Because monosomies are either lethal or unstable, studies of the effects of aneuploidy on cellular physiology have mainly focused on the consequences of one aspect of aneuploidy, i.e. the gain of extra chromosomes. In summary, in addition to being facile and quick, a major advantage of these approaches is that different aneuploid strains can be generated in an isogenic background, making it possible to assess the effects of aneuploidy on cellular physiology, in the absence of other genomic alterations.
3 Aneuploidy Hampers Cellular Proliferation
A simple hypothesis that could explain the detrimental effects of aneuploidy during human development and the uncontrollable proliferation of cancer cells is that aneuploidy leads to increased fitness and promotes proliferation at the cellular level. During development, these properties would allow aneuploid cells to circumvent regulatory mechanisms, such as senescence and apoptosis, required for normal development. In cancer, increased cellular proliferation and resistance to death are indeed hallmarks of the disease (Hanahan and Weinberg 2011). However, studies in aneuploid yeast suggest that the opposite occurs, at least under normal (non-stress) conditions; that is, without exceptions, acquisition of an extra chromosome inhibits cell cycle progression and lowers cell viability, independent of the identity of the gained chromosome (Fig. 1). Impaired proliferation of aneuploid yeasts is not simply due to the presence of extra DNA, as yeast strains harboring yeast artificial chromosomes (YAC) of comparable sizes to the yeast chromosomes harboring human or mouse DNA do not show cell cycle delays (Torres et al. 2007). These results indicate that the presence of the extra yeast genes and their products is responsible for the proliferation defects. Interestingly, the degree to which proliferation is affected in aneuploid yeast cells correlates with the number of open reading frames (ORF) encoded by the extra chromosome, underscoring the idea that aneuploidy is a problem of genomic imbalance. Earlier studies in plants and flies showed that the detrimental effects of acquiring extra chromosomes correlate with the size of the extra genomic material (Blakeslee et al. 1920; Lindsley et al. 1972). In humans, Chromosome 21 encodes the least number of proteins compared to other autosomes, possibly explaining why trisomy 21 is the only viable one.
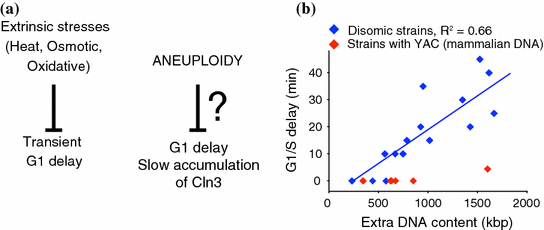
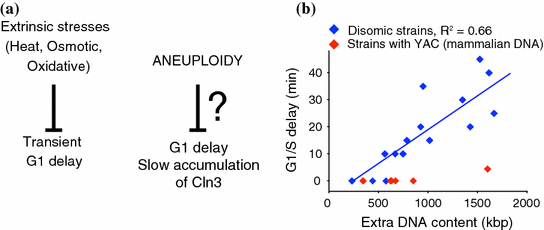
Fig. 1
Aneuploidy causes cell cycles delay in manner similar to extrinsic stresses (a), the G1 delays observed in aneuploid yeast strains correlate with the size of the extra chromosome they harbor (b)
The molecular mechanisms by which aneuploidy hampers cellular proliferation are not well understood. Studies of disomic yeasts synchronized in G1 revealed that the transition through G1/S-phase during cell cycle progression was the one most affected by aneuploidy (Torres et al. 2007). Subsequent studies of small G1 cells isolated by elutriation showed that disomic yeasts display a cell cycle entry delay due to a slowed accumulation of the G1 cyclin CLN3 (Thorburn et al. 2013). Recently, acetyl-CoA was shown to control CLN3 transcription by promoting the acetylation of histones present in its regulatory region raising the possibility that altered metabolism may be responsible for the cell cycle delays in aneuploid yeast (Shi and Tu 2013). However, in aneuploid yeast cells CLN3 transcript levels do not seem to be affected, indicating that posttranscriptional mechanisms are responsible for the delayed Cln3 protein accumulation (Thorburn et al. 2013). It is noteworthy, that independent of the nature of extrinsic cellular stresses, yeast cells usually arrest in G1 (Belli et al. 2001; Lee et al. 1996; Rowley et al. 1993). This implies that the same molecular mechanisms that arrest yeast cells in response to extrinsic stresses may play a role in mediating cellular responses to aneuploidy. Several pathways and factors control Cln3 protein levels during G1 including nutrients, Map kinase and Ras-cyclic AMP signaling pathways (Johnson and Skotheim 2013). Which of these pathways is/are affected by aneuploidy remains to be investigated. The effects on cell cycle progression in aneuploid yeast cells isolated from random meiosis have not been studied, yet 38 of such strains proliferate slower than their euploid counterparts (Pavelka et al. 2010).
Studies of aneuploid yeasts suggest that in the absence of other genomic alterations, aneuploidy inhibits proliferation of mammalian cells. Consistent with this hypothesis, embryonic fibroblasts from Down syndrome patients proliferate slower and accumulate less biomass than fibroblasts obtained from healthy individuals (Rosner et al. 2003; Segal and McCoy 1974). Similarly, primary mouse cells and immortalized human cell lines harboring an extra chromosome show impaired proliferation (Stingele et al. 2012; Williams et al. 2008). Interestingly, in a study that induced aneuploidy in near diploid cancer cell line by chromosomal missegregation, cell cycle arrest occurred in G1 in a manner dependent on p53 (Thompson and Compton 2010). This implied a possible role for this tumor suppressor, whose protein levels are induced by a myriad of cellular stresses (Zilfou and Lowe 2009), in the anti-proliferative effects of aneuploidy.
In summary, aneuploidy seems to inhibit cell cycle progression from yeast to humans, similar to other cellular stresses. However, the specific signaling pathways mediating this effect still remain to be explored. Nevertheless, a major implication of the aneuploidy studies described above is that other genetic alterations or environmental factors must exist to allow cancer cells to overcome the aneuploidy-induced growth inhibitory effects.
4 Transcriptional Responses to Aneuploidy
The fact that yeast strains harboring YAC with human or mouse DNA do not show cell cycle defects indicates that expression of the yeast genes present on the extra chromosome is responsible for the hampered proliferation of aneuploid yeasts. Indeed, several studies have reported an increase in the levels of mRNAs encoded by the extra chromosomal genes in aneuploid cells (Fig. 2). Gene expression analysis of 17 aneuploid strains generated by chromosome transfer and five aneuploid strains recovered from random meiosis showed that on average, levels of mRNAs encoded by genes on the additional chromosome proportionally increase with gene copy number (Pavelka et al. 2010; Torres et al. 2007). The correlation between gene copy number and mRNA expression is so strong that gene expression profiling alone can reveal changes in chromosome numbers (Fig. 2). For example, gene expression analysis of hundreds of yeast strains harboring a single gene deletion revealed widespread aneuploidy among them (22 of 290) (Hughes et al. 2000). Several studies also indicate that the correlation between increased gene copy number leading to changes in gene expression is also true in trisomic mouse embryonic fibroblasts, trisomic human cell lines and aneuploid cancer cells (Crawley and Furge 2002; Hyman et al. 2002; Stingele et al. 2012; Williams et al. 2008). Despite this correlation, the possibility that mRNA levels of a small number of genes do not scale up with gene copy number and are subject to dosage compensation through gene silencing or posttranscriptional mechanisms remains to be investigated. While genome-wide approaches are very powerful at revealing general patterns of gene expression, a more gene-centric approach will be necessary to unveil the identity of such genes. Nonetheless, a major conclusion from these studies is that while dosage compensation mechanisms play a key role in regulating gene expression of sex chromosomes, such mechanisms do not seem to be activated upon acquisition of an extra copy of an autosome. In support of this hypothesis, the introduction of a single copy of the gene XIST, X-inactivation gene, on Chromosome 21 was shown to silence transcription and suppress several phenotypes, including deficits in proliferation in pluripotent stem cells of down syndrome patients (Jiang et al. 2013).
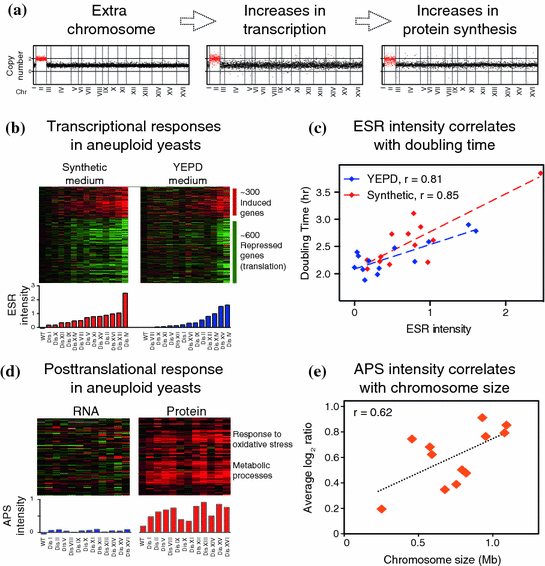
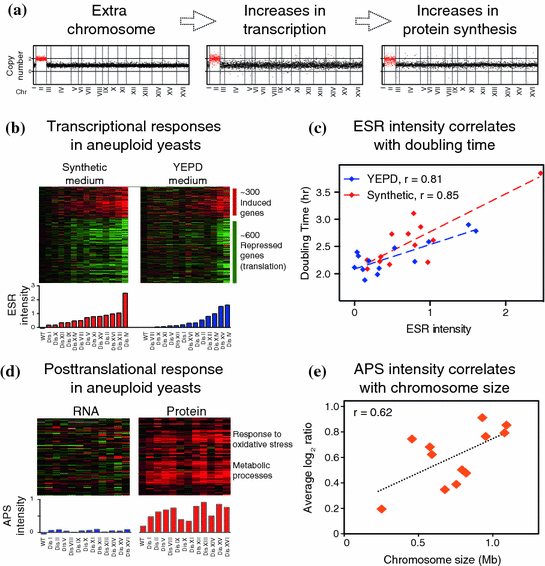
Fig. 2
a Relative DNA, mRNA and protein content of cells harboring an extra copy of chromosome II (disome II). Data obtained from Torres et al. (2007) and Dephoure et al. (2014). b Aneuploid elicits a transcriptional response similar to the environmental stress response. c The intensity of this response correlates with proliferation rate (r = Pearson correlation). Data obtained from Torres et al. (2007). d Aneuploid cells show an aneuploidy-specific protein signature (APS) associated with altered metabolism and redox stress. This signature is mediated by posttranslational mechanisms and its intensity correlates with the size of the extra chromosome present in aneuploid yeast strains (r = Pearson correlation)
In addition to changes in mRNA resulting from increased gene copy number, aneuploidy elicits a transcriptional response similar to that of cells exposed to cellular stresses, such as heat shock, oxidative or osmotic stress, among others (Gasch et al. 2000) (Fig. 2b). This transcriptional signature referred to as the environmental stress response (ESR) encompasses approximately 600 downregulated and 300 upregulated genes. The common denominator between cellular responses to extrinsic stresses is transient cell cycle arrest. In fact, gene expression profiling of wild-type yeast grown in chemostats, where the proliferation rate can be adjusted by changing dilutions rates of different nutrients, showed a significant overlap between genes that respond to stress and those whose expression levels change as a function of proliferation rate (Brauer et al. 2008). For example, ribosomal genes are downregulated upon several stresses and change their expression levels as a function of proliferation rates. This raises the question of whether aneuploid cells display a common gene expression signature due to their impaired proliferation or to induced cellular stress. Because stress responses are tightly coupled with cell cycle arrest this is a difficult question to answer. Nonetheless, there is a strong correlation between the intensity of the ESR in disomic yeasts, measured as the weighted average of up-regulated and down-regulated genes, and cell cycle delays (Fig. 2c). Comparison of gene expression changes in disomic strains grown in selective medium versus rich medium indicates that the slow growth signature is ameliorated in rich media (Fig. 2b, c). Because the differences in proliferation rates between disomic strains and wild-type cells are less in rich media than in selective medium, the slow growth expression signature seems to be partly a function of proliferation. Consistent with this hypothesis, euploid cells harboring gene mutations that confer proliferation defects show similar gene expression patterns (Torres et al. 2007). The slow growth expression signature is also present in several aneuploid strains isolated from random meiosis, which proliferate slower than the euploid cells (Pavelka et al. 2010; Sheltzer et al. 2012). Importantly, similar patterns of gene expression have been observed in aneuploid fission yeast, plants, mouse, and human cells (Sheltzer et al. 2012). Altogether, these studies indicate that aneuploidy hampers cellular proliferation resulting in transcriptional changes similar to those observed in cells affected by extrinsic cellular stresses or in cells that experience hampered proliferation.
5 Aneuploidy Alters Proteome Content of The Cell
A key question that arises from transcriptional analysis is whether changes in mRNAs proportionally translate into changes in protein levels. Understanding how changes in gene expression affect the proteome content of aneuploid cells is of particular importance, as it could reveal novel cellular responses to aneuploidy mediated by posttranscriptional mechanisms.
Quantitative proteome analyses indicate that on average, increases in gene copy number lead to proportional increases in protein levels in all aneuploid strains analyzed (Fig. 2a). Importantly, these results are independent of the method used to generate the aneuploid strains, the growth conditions implemented, or mass spectrometry method utilized to quantify the proteomes. Utilizing stable isotope labeling of amino acids in cell culture (SILAC) and liquid chromatography-mass spectrometry, quantification of protein abundances in 12 different disomic strains revealed quantitative information for ~70–80 % of all verified open reading frames (ORFs) relative to wild-type cells (Dephoure et al. 2014). Specifically, it was shown that on average, the levels of proteins encoded by the additional chromosome increase by an approximate 2-fold. These results were reproduced when aneuploid cells were grown in rich media and their proteome quantified utilizing a different mass spectrometry approach referred to as isobaric tandem mass tag (TMT)-based quantitative mass spectrometry (Dephoure et al. 2014). A similar conclusion was reached using yet a another technique, multidimensional protein identification technology or MudPIT, to analyze the proteome content of five aneuploid cells isolated from random meiosis (Pavelka et al. 2010). Altogether, these analyses indicate that a major consequence of gaining an extra chromosome is increased protein synthesis. Therefore, gaining an extra chromosome leads not only to cellular imbalances due to the extra activity of the proteins encoded on the duplicated chromosome but also to increased burden on the protein quality control pathways including protein synthesis, folding and turnover (see below). In addition, because much of ATP utilization in the cell is devoted to protein synthesis, aneuploidy causes a significant demand for energy, leading to a disruption in cellular metabolic processes, independent of the identity of the chromosome gained.
6 Subunits of Macromolecular Complexes are Significantly Attenuated in Aneuploid Cells
Proteome quantification of disomic yeast strains revealed that despite the general correlation between increased gene copy number, mRNA and protein levels, approximately 20 % of the proteins encoded by the genes located on duplicated chromosomes do not scale up with copy number of their corresponding genes (Fig. 3) (Dephoure et al. 2014). Neither growth conditions nor the quantitative approach utilized affected the degree of attenuation, because quantification of protein levels in aneuploid cells grown in selective or rich media, that were analyzed via two distinct mass spectrometry approaches showed reproducible results. In addition, proteome analysis of five aneuploid strains generated by random meiosis showed that 23–38 % of duplicated proteins were significantly attenuated (Fig. 3b). These results indicate that although acquisition of an extra chromosome leads on average, to 2-fold increases in protein levels of the added genes, a large and statistically significant number of proteins do not increase proportionally with copy number. Interestingly, analysis of the identity of the attenuated proteins revealed that most of the attenuated proteins are subunits of macromolecular complexes. Enrichment of subunits of macromolecular complexes among the attenuated proteins was observed in every aneuploid strain analyzed, including 12 disomic strains generated by chromosome transfer and five aneuploid strains generate by random meiosis.
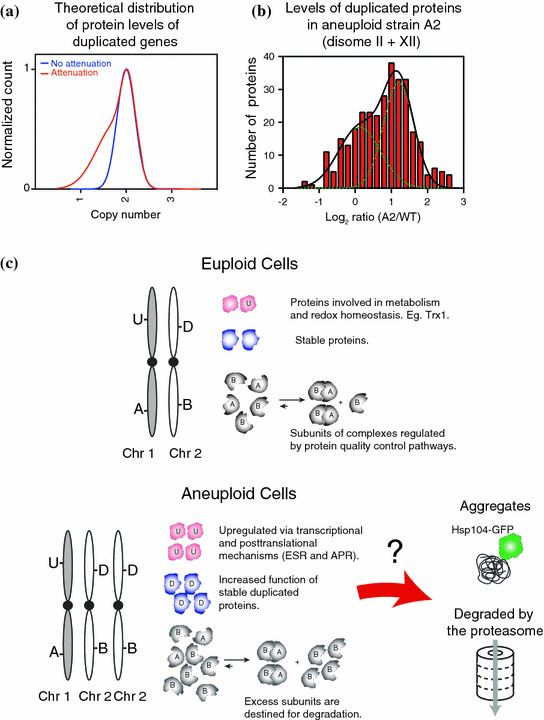
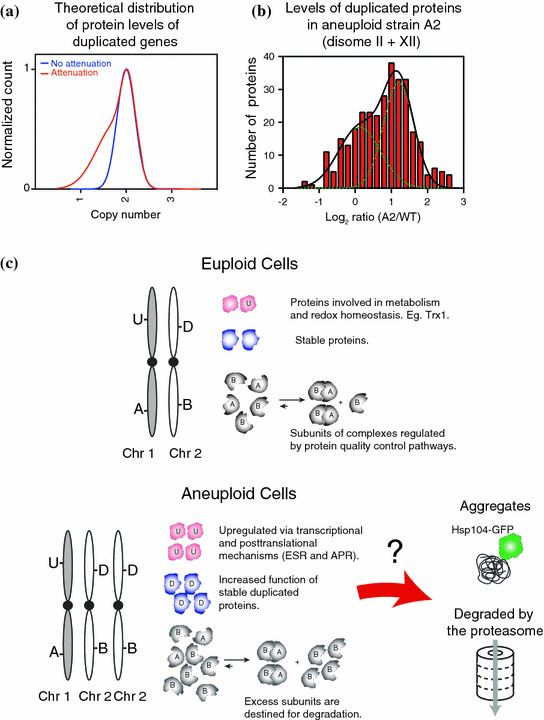
Fig. 3
a Theoretical distribution of protein levels upon gaining an extra chromosome. b Distribution of the levels of duplicated proteins in aneuploid strain A2 (Pavelka et al. 2010). c Schematic of the consequences of increased protein synthesis in aneuploid cells. U represent proteins that are upregulated by transcriptionally (ESR) or posttranslationally (APS) mechanisms; D represent proteins whose levels proportionally correlated with gene copy number; A and B represent subunit of complex AB. Excess proteins could either form protein aggregates or be degraded by protein degradation pathways
Protein attenuation of subunits of macromolecular complexes is mainly mediated by posttranslational mechanisms, most likely protein degradation. Indeed, ribosomal footprinting analysis of two disomic strains demonstrate similar translation efficiencies for the proteins encoded by the duplicated genes, independent of whether the cellular protein levels were attenuated (Dephoure et al. 2014; Thorburn et al. 2013). Nevertheless, translational control may play a role in the attenuation of a small subset of genes and remains to be investigated. In summary, these results provide direct evidence that a major consequence of aneuploidy is increased protein degradation.
A hypothesis that could explain the attenuation of the protein levels of duplicated genes is that protein stability of individual subunits is dependent upon their ability to be incorporated into multi-subunit molecular assemblies (Fig. 3c). Cells have evolved several mechanisms that facilitate complex assembly such as co-transcriptional regulation and dedicated chaperone systems that help stabilize unstable subunits so as to prevent their degradation [e.g. (Burgess and Zhang 2013; Kunjappu and Hochstrasser 2014; Xie and Varshavsky 2001)]. Recently, analysis of protein synthesis in Escherichia coli and yeast showed that subunits of macromolecular complexes are produced in equimolar amounts through similar synthesis rates (Li et al. 2014). Acquisition of an extra chromosome disrupts such modes of regulation, leading to the production of excess subunits that cannot be assembled into stable complexes, and are therefore degraded. Consistently, analysis of the ribosome and the nucleosome subunits indicate that these proteins are short-lived unless assembled into their proper complexes (elBaradi et al. 1986; Gunjan and Verreault 2003; Meeks-Wagner and Hartwell 1986; Tsay et al. 1988). In aneuploid yeast, analysis of protein levels of duplicated subunits of several macromolecular complexes showed a large range of subunit stabilities. Remarkably, almost every complex analyzed contains at least one or more subunits that are degraded when produced in excess. Conversely, most macromolecular complexes with the exception of the nucleosome and ribosome contain at least one subunit that appears to be stable on its own. These results indicate that for most complexes, a stable scaffold protein may be required for complex assembly. Importantly, attenuation of subunits of macromolecular complexes was also observed in immortalized human cell lines harboring extra chromosomes (Stingele et al. 2012). These results indicate that, from yeast to humans, acquisition of extra chromosomes leads to increased synthesis of unstable proteins that can create a burden on the protein folding and degradation pathways of the cell.
7 Cellular Responses to Aneuploidy Are Mediated by Transcriptional and Posttranscriptional Mechanisms
Proteome analysis of aneuploid strains revealed that transcriptional changes in aneuploid yeast associated with the ESR/slow growth signature translate into changes in protein levels. As a result, downregulation of ribosomal genes, which is a major part of the ESR/slow growth response, leads to lowered ribosome levels in the aneuploid strains. Ribosomal footprinting of 2 disomic strains do not show any signs of impaired translation. Therefore, the decreased ribosomal protein levels observed in aneuploid cells while maintaining seemingly normal efficiencies of protein synthesis may account for the hypersensitivity of aneuploid cells to drugs that target the translation machinery.
In addition to changes in protein levels resulting from transcriptional changes, clustering analysis revealed an additional signature of upregulated proteins common among all aneuploid yeast strains analyzed (Fig. 2d, e) (Dephoure et al. 2014). This novel protein signature, termed the aneuploidy-specific protein signature (APS), was observed in 12 disomic strains generated by chromosome transfer and five aneuploid strains obtained from random meiosis. Gene ontology enrichment analysis revealed that the APS proteins are associated with cellular responses to oxidative stress and metabolic processes such as amino acid biosynthesis and cellular bioenergetics. Interestingly, the intensity of the APS as measured by the average increase of its 92 proteins, correlates with the size of the additional chromosome, indicating that it may be a direct consequence of the cellular imbalances resulting from the acquisition of an extra chromosome. Surprisingly, the corresponding mRNA transcripts for most of the upregulated proteins are not increased indicating that the upregulation in protein levels is mediated posttranscriptionally. These results indicate that aneuploidy causes alterations in redox homeostasis and metabolism (discussed below). An important question that remains to be investigated is to identify the molecular mechanism by which the APS proteins are upregulated. Ribosome footprinting analysis of disomic strains for chromosome V and XVI suggest that posttranslational mechanisms mediate upregulation of the APS proteins as no increases in translation efficiencies were detected. Therefore, posttranslational modifications may certainly play a role as most of the 92 APS proteins have been shown to be ubiquitinated or phosphorylated. Lastly, these results highlight the importance of proteomic studies to potentially identify novel biomarkers of aneuploid cells not detected by transcriptional analysis alone that could be exploited therapeutically for the detection as well as targeting of aneuploid cancer cells (Hanash and Taguchi 2010).
8 A Major Consequence of Aneuploidy is Increased Burden on the Protein Quality Control Pathways
Gaining extra chromosomes lead to increased protein synthesis, folding and turnover. As a result, aneuploid cells experience an increased burden on the protein quality control pathways leading to proteotoxic stress. Several lines of evidence support this hypothesis. Aneuploid cells are hypersensitive to high temperature, to translation inhibitors such rapamycin and cycloheximide, to the Hsp90 chaperone inhibitor geldanamycin, and to the proteasome inhibitor MG132. Introducing a conditional loss-of-function allele of the proteasome lid subunit Rpn6 encoding gene is synthetically lethal in two disomic strains and significantly decreases the proliferative abilities of all several other disomic strains. In addition, aneuploid yeast cells are prone to aggregate formation of endogenous proteins as well as ectopically expressed hard-to-fold proteins (Oromendia et al. 2012). Most of the signs of proteotoxic stress are present in aneuploid strains generated by chromosome transfer or recovered from random meiosis. Importantly, the identity of the endogenous proteins that are prone to aggregation is unknown. An interesting hypothesis that is yet to be investigated is that aggregate formation caused by increased synthesis of proteins encoded by the duplicated genes could lead to the sequestration of other abundant cellular proteins with essential housekeeping functions, thereby causing a disruption in cellular homeostasis (Olzscha et al. 2011).
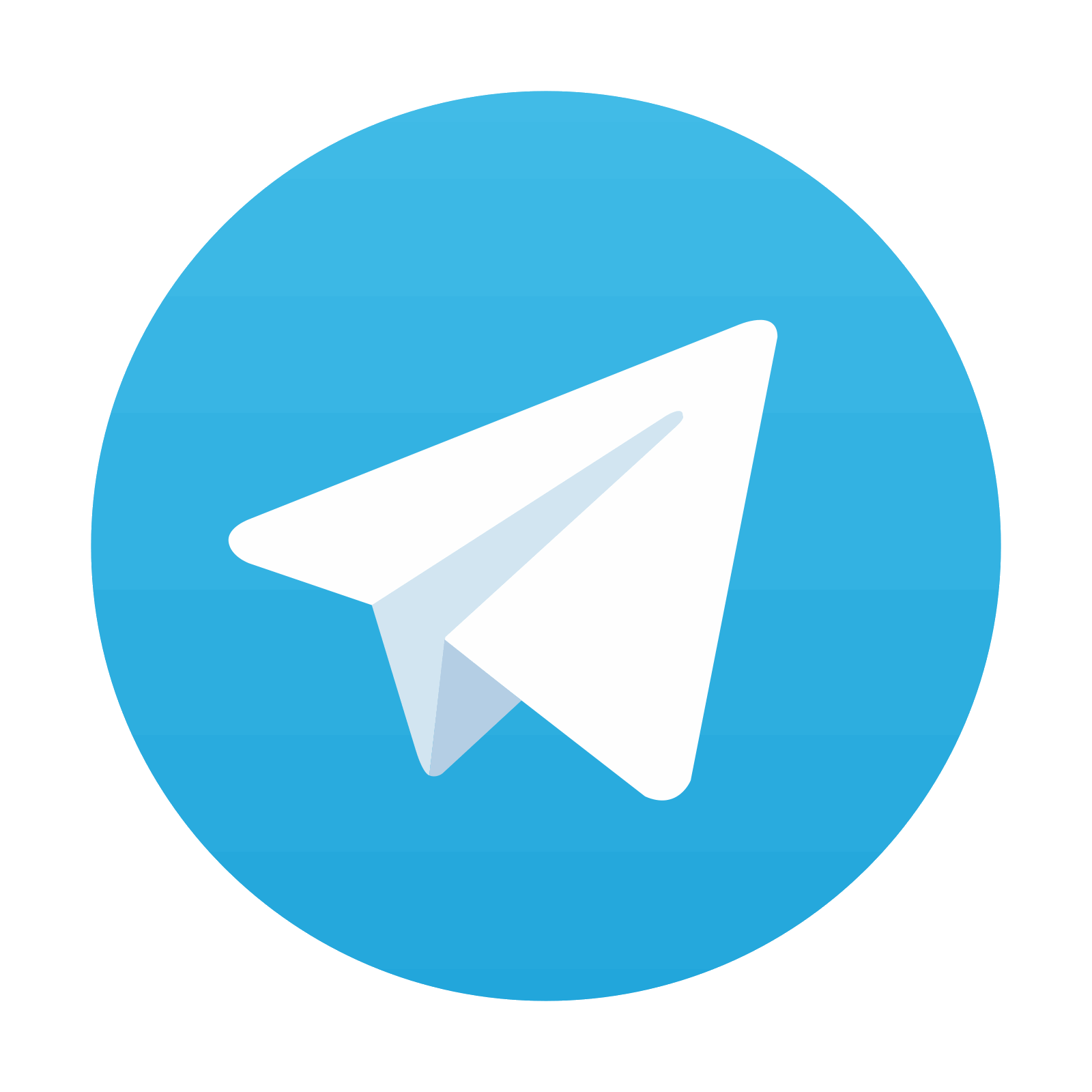
Stay updated, free articles. Join our Telegram channel
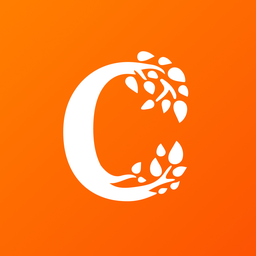
Full access? Get Clinical Tree
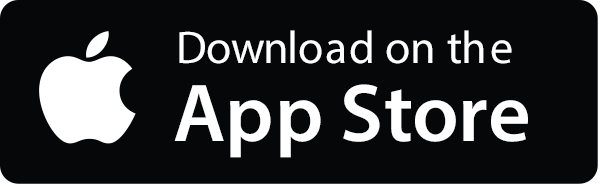
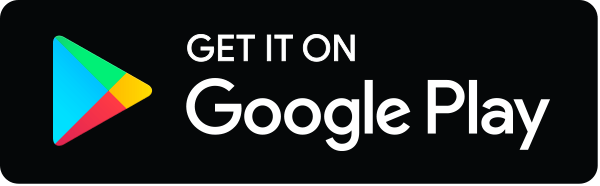