Fig. 18.1
Signs of responding vertebral metastases visible on T1w MR imaging (Modified from Lecouvet et al. [31])
- 1.
Shrinkage or progressive fading of the marrow signal intensity abnormalities
- 2.
Development of “fatty halo sign” (hyperintense on T1w), which represents healing
- 3.
Increasing of the intralesional calcified component within correspondent dimensional stability of the lesion
Conversely, possible signs of evolution and/or persistence of active tumor tissue are (Fig. 18.2):


Fig. 18.2
Signs of progressing vertebral metastases visible on T1w MR imaging (Modified from Lecouvet et al. [31])
- 1.
Onset of new marrow signal intensity abnormalities
- 2.
Persistence of noncalcified T1w hypointense and STIR hyperintense foci in a dimensionally stable lesion
- 3.
Development of “cellular halo” (hypointense on T1w and hyperintense on STIR images) at the periphery of a calcified lesion [30]
However, stability in size/appearance of a noncalcified metastasis or dimensional increase of a completely calcified lesion has to be considered as indeterminate signs because they may both be associated with the presence of controlled but still active disease or, on the contrary, “cured” disease with persistence of “scar” tissue [31, 32]. In such cases and when the calcified component is not yet predominant, diffusion-weighted imaging (DWI) will be possibly helping. DWI detects changes in water diffusion that occur when the normal fatty marrow is replaced with highly dense cellularity which restricts normal water movements among cell membranes, so providing morphologic and functional information on lesions. Reconstructed maximum intensity projection (MIP) images of WB-DWI show noncalcified metastatic lesions as high signal intensity foci providing an “at a glance” evaluation of the probably active metastatic involvement [33]. In Table 18.1 are resumed the performances of radiological devices for the detection of bone metastases.
Table 18.1
Performances of radiological devices for the detection of bone metastases
Author (ref) | No. of pts | Sensitivity (%) | Specificity (%) | PPV (%) | NPV (%) | Accuracy (%) | |
---|---|---|---|---|---|---|---|
Conventional radiology | Lecouvet et al. [34] | 66 | 63 | 64 | 100 | 70 | – |
Ketelsen et al. [35] | 14 | 58.6 | – | – | – | – | |
Lecouvet et al. [36] | 100 | 86 | 98 | 98 | 87 | – | |
CT | Luboldt et al. [37] | 15 | 67 | – | – | – | – |
MRI axial skeleton only | Lecouvet et al. [36] | 66 | 100 | 88 | 100 | 100 | – |
Luboldt et al. [37] | 15 | 93 | – | – | – | – | |
WB-MRI with DWI | Luboldt et al. [37] | 15 | 100 | – | – | – | – |
Ketelsen et al. [35] | 14 | 96.4 | – | – | – | – | |
Venkitaram et al. [38] | 39 | 70 | 100 | 100 | – | – | |
Wang et al. [39] | 49 | 100 | 87.2 | – | – | – | |
Gutzeit et al. [40] | 35 | 91 | 99 | 97 | 97 | – | |
Mosavi et al. [41] | 49 | 100 | 98 | 83 | 100 | 98 | |
Lecouvet et al. [34] | 100 | 98 | 98 | 98 | 98 | – | |
Stecco et al. [42] | 23 | 80 | 98.2 | – | – | – |
Comparison of consecutive examinations delivers an easy and generally non-ambiguous evaluation of the disease response or progression under therapy (Fig. 18.3).


Fig. 18.3
A 6-month follow-up imaging using WB-MRI in 69-year-old man with long-term bone diffuse metastatic disease and new signs of progression during antiandrogenic therapy. (a, b) Coronal T1w images show long-term calcified metastases as multiple hypointense foci involving vertebrae, ribs, and left femur in (a) and appearance of both new bone marrow hypointense foci (femurs and iliac bones) and low-signal intensity tissue adjacent to older ones (e.g., see L2 and left femur in (b)). (c, d) DWI images with 3D radial-MIP (maximum intensity projection) reconstruction identify progression of disease from (c) to (d) as new appearance of hyperintense bone foci representing tissue with restricted diffusion due to high cellularity (i.e., see left femur, thoracic and lumbar vertebrae). Remaining bone metastases are not clearly seen on DWI, representing false-negative findings due to advanced sclerotic changes inside the lesions
DWI also allows the calculation of the apparent diffusion coefficient (ADC, units * 10−3 mm2/s) values of the lesions, representing a quantitative analysis used in monitoring over time the response to chemotherapy. It has been in fact shown that ADC values increase within prostate cancer metastases treated with antiandrogen therapy as early as 1 month after treatment initiation [43, 44].
However, both visual and quantitative analysis methods provided by DWI may present pitfalls. In particular, predominantly calcified metastases remain an issue representing false-negative visual findings, and the interpretation of changes in ADC values is indeed complex, mainly because of tumor and response heterogeneity. Newer analysis methods (ADC parametric response or functional diffusion map) taking spatial information and tumor heterogeneity into account and enabling voxel-by-voxel follow-up of treatment-induced changes seem to overcome these pitfalls [44, 45]; however, further validation by literature is needed. Furthermore, optimization of hardware, sequences and signal analysis, and definite standardization of acquisition method are also necessary to improve the reliability of the results in the future. In Table 18.2 are reassumed the pros and cons of the radiological examinations employed for the detection of bone lesions.
Table 18.2
Pros and cons of radiological examinations
Pros | Cons | |
---|---|---|
Radiography | 1. High availability | 1. Low diagnostic accuracy |
2. Low cost | 2. Not all bones can be screened | |
3. Easy for the patient to undergo | 3. Equivocal and late response to therapy | |
4. Allows assessment of complications (i.e., fractures) | ||
CT | 1. Allows fine bone detail assessment and smaller lesion characterization | 1. High radiation dose |
2. Allows evaluation of entire skeleton | 2. Limited ability in the assessment of therapy response | |
MRI with WB and DWI acquisitions | 1. Highest diagnostic performance in detection and characterization of bone lesions | 1. Advanced diagnostic techniques only available in diagnostic imaging center of excellence |
2. Possible role in the assessment of therapeutic response | 2. Longer duration of examination, higher costs |
18.2.5 Nuclear Medicine Imaging
Nuclear medicine offers different options for the detection of bone metastases in PC patients: (a) bone scan (BS) as a planar or tomographic imaging (i.e., single-photon emission tomography, SPET) and (b) PET/CT with 18F-fluoride or 18F-fluorodeoxyglucose (FDG) or 11C/18F-choline or 11C-acetate or 68Ga-prostate-specific membrane antigen (PSMA) or 18F-anti-1-amino-3-18F-fluorocyclobutane-1-carboxylic acid (FACBC). Each imaging technique shows a specific mechanism of action to detect bone metastases due to a different uptake and metabolism of the radiopharmaceuticals as extensively reported in Chaps. 7, 8, and 9; therefore, they are associated with different diagnostic performances, mainly based on the type of skeletal lesion (i.e., osteoblastic vs. osteolytic vs. bone marrow invasion) [46–48].
Planar BS using 99mTc-diphosphonates is the standard technique to detect skeletal metastasis from PC as it is widely available, relatively inexpensive, and highly sensitive. However, the mechanism of uptake of 99mTc to a suitable phosphonate images the sites of blastic or mixed lesions, missing areas where calcium deposit is missing. This is the reason why BS shows low specificity (falsely positive in case of benign lesions, prior trauma, and arthritis) and flare phenomena. Therefore, an osteoblastic response that occurs as a result of bone healing/flare response during systemic treatments can significantly alter its diagnostic performance and makes very difficult the clinical interpretation of scintigraphic findings. Moreover, in a large retrospective analysis, bone metastases were found in less than 1 % of patients with PSA of <20 ng/mL, therefore yielded a negative predictive of 99.7 % [49]. Although the introduction of tomographic imaging, such as SPET and SPET/CT, has overpassed some limits of BS, these modalities are not able to cover the entire body of patients, and as reported by Hillner et al. [50], SPET/CT is not currently clinical practice. An interesting possibility offered by BS is the calculation of bone scan index (BSI), than reflecting the extend of metastatic disease [51, 52]. This approach seems to be very interesting, since its measurement can be also automated; nevertheless, this technique was not successful in the clinical routine and remains cumbersome to be adopted [52] (Fig. 18.4).


Fig. 18.4
Serial bone scans in patient with prostatic adenocarcinoma (Gleason score, 5 + 4). (Left) First bone scan performed for a biochemical recurrence of disease, after radical treatment. (Middle) Second scan, after the administration of bipolar androgen therapy (BAT). (Right) Third bone scan, after the administration of a further hormonal therapy
Even with persisting high costs, PET represents an efficient modality for whole-body scanning in a reasonably short time. With the increasing availability of PET/CT scanners, the possibility of obtaining more detailed and precise CT anatomic localization of PET-directed metabolic abnormalities of tumor lesions, especially in skeletal diseases, has become a clinical reality. Nowadays, a lot of radiopharmaceutical agents are available for PET/CT imaging, especially for the detection of bone metastases. 18F-Fluoride has the desirable characteristics of high and rapid bone uptake accompanied by very rapid blood clearance, which results in a high bone-to-background ratio in a short time. 18F/11C-Choline and 11C-acetate are able to identify the presence of viable PCa tissue and have shown promising results especially for the early detection of bone marrow infiltration. 18F-FDG was mainly used for the definition of osteolytic lesions [52] but seems to be able to identify the presence of viable cells in osteoblastic ones even if the majority of PC displays a low glycolytic metabolic behavior and does not suggest its current application. In Table 18.3, the performances of nuclear imaging techniques for the detection of bone metastases are reassumed.
Table 18.3
Performances of nuclear medicine modalities for the detection of skeletal lesions in prostate cancer patients
Authors (ref) | No. of pts | Sensitivity (%) | Specificity (%) | PPV (%) | NPV (%) | Accuracy (%) | |
---|---|---|---|---|---|---|---|
99mTc-phosphonates Bone scan | Garcia et al. [54] | 91 | 65.4 | 38.5 | 86.4 | 15.6 | 61.5 |
Poulsen et al. [55] | 50 | 50.8 | 82.2 | 86.4 | 42.9 | 60.6 | |
Even-Sapir et al. [47] | 44 | 57 | 57 | 59 | 55 | – | |
Iagaru et al. [56] | 18 | 87.5 | 80 | – | – | – | |
Damle et al. [57] | 72 | 96.9 | 41.2 | 75.6 | 87.5 | 77.5 | |
Palmedo et al. [58] | 97 | 96.4 | 75.3 | 98.1 | 61.4 | – | |
Withofs et al. [59] | 10 | 66.7 | 81.6 | 53.3 | 88.6 | 78 | |
Takesh et al. [60] | 37 | 89.3 | – | – | – | – | |
99mTc-phosphonates SPET | Even-Sapir et al. [47] | 44 | 78 | 67 | 72 | 74 | – |
Palmedo et al. [58] | 97 | 96.4 | 63.7 | 97.8 | 51.9 | ||
99mTc-phosphonates SPET/CT | Palmedo et al. [58] | 97 | 96.4 | 94.2 | 98.5 | 87.1 | |
18F-Fluoride PET/CT | Poulsen et al. [55] | 50 | 93.1 | 54 | 81.8 | 77.9 | 81 |
1. Even-Sapir et al. [47]b | 44 | 100 | 62 | 74 | 100 | – | |
Even-Sapir et al. [47]c | 44 | 100 | 100 | 100 | 100 | – | |
Beheshti et al. [61] | 38 | 81 | 93 | – | – | 86 | |
Langsteger et al. [62] | 42 | 91 | 83 | – | – | 88 | |
Iagaru et al. [56] | 18 | 100 | 100 | – | – | – | |
Damle et al. [57] | 72 | 100 | 70.6 | 86.5 | 100 | 65.4 | |
Withofs et al. [59] | 10 | 100 | 89.5 | 75 | 100 | 92 | |
18F-FDG PET/CT | Iagaru et al. [56] | 18 | 55.6 | 80 | – | – | – |
Damle et al. [57] | 72 | 71.9 | 100 | 100 | 65.4 | 81.6 | |
18F-Choline PET/CT | Beheshti et al. [63] | 70 | 79 | 97 | 84 | – | – |
McCarthy et al. [64] | 26 | 96 | 100 | – | – | – | |
Poulsen et al. [55] | 50 | 84.7 | 91.1 | 95 | 74.9 | 86.8 | |
Beheshti et al. [61] | 38 | 74 | 99 | – | – | 88 | |
Langsteger et al. [62] | 42 | 91 | 89 | – | – | 90 | |
Takesh et al. [60] | 37 | 82.7 | – | – | – | – | |
11C-Choline PET/CT | Fuccio et al. [65] | 25 | 86 | 100 | – | – | – |
Garcia et al. [54] | 91 | 96 | 92.3 | 98.7 | 80 | 95.6 | |
Picchio et al. [66] | 78 | 89 | 98 | 96 | 94 | 95 | |
11C-Acetate PET/CT | Beheshti et al. [67] | a | 81.6a | 98.8a | – | – | – |
The most recent data available in the literature demonstrate a role for radiolabeled choline PET/CT in the assessment of new hormonal therapies, such as enzalutamide [68, 69] or abiraterone acetate [70], and chemotherapy (i.e., docetaxel) [71] (Figs. 18.5 and 18.6). Choline PET/CT findings agree with PSA changes in the majority of patients with progressive disease, during and after therapy. Conversely, the disappearance of uptake does not always correlate with the disappearance of the cancer lesion since it could be due to the effect of a stable or non-metabolically active focus. Moreover, the appearance of new areas of uptake does not always correlate with certain progression due to the well-known phenomenon of flare reaction, whose correct interpretation in BS has been standardized. This issue is an open area of debate.



Fig. 18.5
A 73-year-old patient with an advanced prostate cancer underwent serial PET/CT scans before starting and during abiraterone acetate for monitoring the response to therapy. A correlation between metabolic imaging and biochemical values was found

Fig. 18.6
A 65-year-old man, with a prostatic adenocarcinoma (pT2bN0M0; GS 3 + 4). PET/CT was performed before (up) and after (down) the administration of docetaxel and zoledronic acid. At the time of the second PET/CT scan, PSA was 6.4 ng/ml. The images revealed an increase of uptake in the pelvis compatible with a progression of disease
18.2.5.1 Flare Phenomenon and Nuclear Medicine Modalities
There some fundamental differences in diagnostic imaging, when they are used to detect cancer lesions and to evaluate changes during any treatment. Nuclear medicine modalities can mainly provide metabolic information and trace some pathologic processes depending on the different employed radiopharmaceuticals, like those for cancer cells, for inflammatory cells, for bone, and for others. This is the reason why, in particular for bone metastases, radiopharmaceuticals have to be differentiated in two main groups: “bone-targeting agents” (99mTc-phosphonates and 18F-Fluoride) and “cancer-targeting agents” (F18/C11 choline, 18F-FDG, 68Ga-PSMA, 18F-FACBC) (Fig. 18.7), according to the biological processes for their uptake’s mechanism. Bone-targeting agents mimic the Ca++ ions path and are able to show the bone remodeling that surrounds bone metastases. On the contrary, cancer-targeting agents enter into the glycolytic metabolism of cancer cells, or participate to the phospholipid turnover of membranes, or again bind the membrane receptors of kallikrein, androgen, etc.


Fig. 18.7
Mechanism of bone metastasis formation and different targets (bone matrix and cancer cells) of the radiopharmaceuticals used in nuclear medicine to image prostate cancer bone metastases. AR androgen receptor, ET1 endothelin 1, 18F-FACBC 18F-anti-1-amino-3-fluorocyclobutane-1-carboxylic acid, 18F-FDG 18F-2-deoxy-2-fluoro-D-glucose, 18F-FDTH 18F-fluoro-5α-dihydrotestosterone, 18F-FMAU 18F-2’-fluoro-5-methyl-1-β-D-arabinofuranosyluracil, 68Ga-PSMA 68Ga-labeled prostate-specific membrane antigen, OP osteoprotegerin, PTHrp parathyroid hormone-related protein, RANKL receptor activator of nuclear factor kappa-B ligand, UPA urokinase-type plasminogen activator (From Bombardieri et al. [48])
Prostate cancer bone metastases are imaged by both groups of radiopharmaceutical. However, in accordance with the mechanism of uptake, the behavior of radiopharmaceuticals can be different and therefore is associated with diverse imaging results. Some authors describe an increase of radiopharmaceutical uptake within few weeks after the beginning of the therapy that is correlated with an incorrect interpretation of the imaging and therefore with an erroneous sign of non-response [72, 73]. This “flare” depends on the intense osteoblastic reaction that follows the killing of metastatic localization and determines an increased uptake of radiolabeled phosphonates or fluoride in the crystalline structure of hydroxyapatite. The disappearance of this phenomenon takes usually some months from the start of the drug administration [74]. Based on this metabolic phenomenon, an increased uptake should be interpreted as progression of disease only if new sites of lesion are clearly imaged or a further increase of lesion number is validated by serial scans [75]. The flare phenomenon has been observed in a number of prostate cancer patients under hormonal therapy or treated with chemotherapy [76]. Flare phenomenon has been also observed with cancer-targeting radiopharmaceuticals that are directly incorporated into cancer cells or into the tumor structures [77], particularly for the new class of antihormonal therapies, like abiraterone acetate [70]. Therefore, the biological interpretation should be carefully evaluated because a lot of events can be correlated with the increase of cancer-seeking radiopharmaceutical uptake: (1) inflammatory reaction that follows tumor necrosis and (2) the temporary intensified change of cancer cell metabolism. Again, to overcome these misleading information, it is necessary to evaluate the response to therapy at least after 3 months from the beginning of the treatment, in order to differentiate between disease progression and flare phenomenon. However, some cases of flare phenomenon with 18F-choline PET/CT have been reported in patients undergoing 223Ra dichloride treatment, after the third cycle. In Fig. 18.8, two examples are reported. The mechanisms underlying this process are still unknown; future studies are mandatory.
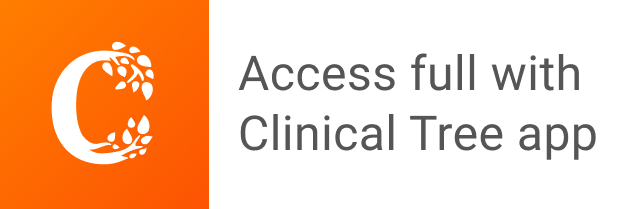