Chapter Outline
THE HEMATOPOIETIC GROWTH FACTORS
ACTIONS OF HEMATOPOIETIC GROWTH FACTORS
Predominantly Lineage Specific HGFs: G-CSF, M-CSF, Il-5, TPO, and EPO
Multilineage Hematopoietic Growth Factors: Il-3 and GM-CSF
Early-Acting Hematopoietic Growth Factors: SCF and FLT3 Ligand
Other Stem Cell Factors: Wnt, Jagged, BMP, and Angiopoietin-Like Growth Factors
Synergistic Factors: Il-1, Il-6, and Il-11
Negative Regulator of Hematopoiesis: Transforming Growth Factor Beta
MOLECULAR BIOLOGY OF HEMATOPOIETIC GROWTH FACTORS
Genomic Analysis of Cytokine and Cytokine Receptor Genes
Structures of Hematopoietic Growth Factor Proteins
Hematopoietic Growth Factor Gene Disruptions
HEMATOPOIETIC GROWTH FACTOR RECEPTORS
CLINICAL USE OF HEMATOPOIETIC GROWTH FACTORS
This chapter offers a review of the anatomy and physiology of normal hematopoiesis that is intended to provide a basis for understanding the marrow failure syndromes described at length in the following chapter. In this chapter we briefly discuss the phylogeny of hematopoiesis and describe marrow anatomy and the egress of recognizable hematopoietic cells from the marrow into the peripheral blood. A more detailed analysis then follows of the cellular bases of erythrocyte, granulocyte-macrophage, and megakaryocyte development, including discussions of the pluripotent stem cell and its niche, the more committed but still undifferentiated progenitor cells, and the differentiated precursors of the mature formed elements of the blood. Much of this chapter is devoted to the interactions of growth factors and the cells that produce them in the upregulation of hematopoiesis. The mechanisms of downregulation of hematopoiesis by cell interactions and cytokines are touched upon here, but they are less well understood despite the fact that they are likely to influence the pathophysiology of aplastic anemia and other marrow failure syndromes.
History *
That “blood is life” was appreciated by Empedocles in the fifth century BC. The theory that the vasculature contains blood, phlegm, black bile, and yellow bile, all revealed when freshly let blood is permitted to separate, is attributed to Polibus, the son-in-law of Hippocrates.
Servetus recognized the systemic and lesser circulations in the sixteenth century. He was burned at the stake, in part because he did not accept the dogma that blood must pass through the intraventricular cardiac septum. (Grant disapproval and, more recently, approval without funding have been substituted for immolation. The effects are not entirely dissimilar.)
In view of the present growth of knowledge of hematology, it is remarkable to realize that the concept of the circulation of the blood was finally established by Harvey in 1628, not even 400 years ago. This began the clinical application of blood transfusion, of which Pepys wrote “it gave rise to many pretty wishes as of the blood of a Quaker to be let into an Archbishop and such like.”
In the mid–seventeenth century, Swammerdam observed red blood corpuscles in the microscope and Malpighi discovered the capillary circulation in the lung and in the omentum. But it was not until the nineteenth century that the source of blood cell production began to be successfully explored. Houston suggested that red cells were derived from leukocytes in the lymphoid system. Zimmerman believed that erythrocytes were derived from platelets, an opinion shared by Hayem. Addison, perhaps not surprisingly, attributed red cell production to the adrenals, and Reikert finally suggested that red cells might be produced in the embryonic liver. In fact, it was not until 1868 that Neumann demonstrated that red cells arise from precursors in the marrow. The modern understanding of the physiology of hematopoiesis then began.
* For an entertaining review from which this precis was in part drawn, see Robb-Smith AHT: The growth of knowledge of functions of the blood, in MacFarlane RG, Robb-Smith AHT, editors: Functions of the blood , Oxford, 1961, Blackwell Scientific Publications. For more details, see also Blood pure and simple by the late Maxwell M. Wintrobe, New York, 1980, McGraw-Hill.
Phylogeny
Much can be learned about the physiology of hematopoiesis from study of the evolution of oxygen transport, a subject reviewed by Lehman and Huntsman.
One of the major advantages of mammalian life over that of invertebrates is the capacity to package large amounts of hemoglobin within cells. This permits the delivery of oxygen to tissues without the enormous increase in oncotic pressure that would be induced by a similar concentration of high–molecular-weight hemoglobin free in the plasma. The renewal rate of red cells is a function of metabolic rate or basal heat production. This is illustrated dramatically in studies of the animal kingdom, ranging from the turtle to the pygmy shrew, and by comparisons of red cell renewal in marmots during periods at ambient and cold temperatures, in rats, and in frogs.
The production of blood cells in bone marrow is a late development in phylogeny. Red cells are found in the coelomic cavity of the worm, and are produced in the kidneys of the goldfish. The influence of oxygen demand on the production of red cells is illustrated by the effects of hyperoxia on bled rats and the behavior of the European eel, one of the few vertebrate forms that ordinarily lacks erythrocytes in its juvenile state. When the adult eel struggles against the current up the rivers of Europe, nucleated cells containing hemoglobin appear in its plasma. This influence of oxygen demand on respiratory pigment production is also illustrated in organisms that do not produce red cells, such as Daphnia , the English water flea, a creature that produces high–molecular-weight hemoglobin in its ovaries when it is exposed to low oxygen tension in stagnant ponds. The discovery of transcription factors that function as oxygen sensors provides a potential molecular explanation for these regulatory mechanisms.
Marrow Anatomy
The active red marrow space of a child is much greater than that of an adult, presumably because the high requirements for red cell production during neonatal life demand the resources of the entire production potential of the marrow. During postnatal life the demands for red cell production ebb, and much of the marrow space is slowly and progressively filled with fat ( Fig. 1-1 ). In certain disease states that are usually associated with anemia, such as myeloid metaplasia, hematopoiesis may return to its former sites in the liver, spleen, and lymph nodes and may also be found in the adrenals, cartilage, adipose tissue, thoracic paravertebral gutters, and even in the kidneys.
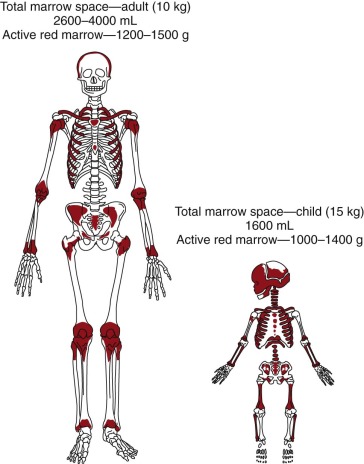
The microenvironment of the marrow cavity is a vast network of vascular channels or sinusoids in which float fronds of hematopoietic cells. This complex area of cell biology and anatomy has been the subject of several reviews. The vascular and hematopoietic compartments are joined by reticular fibroblastoid cells that form the adventitial surfaces of the vascular sinuses and extend cytoplasmic processes to create a lattice on which blood cells are found. The lattice itself is illustrated by reticulin stains of marrow sections ( Fig. 1-2 ). The conformation of the meshwork of reticulin and the location of hematopoietic cells in the network of vascular sinuses are best illustrated by scanning electron microscopy ( Fig. 1-3 ). The fibroblastoid network has two major functions: (1) it provides an adhesive framework onto which the developing cells are bound, and (2) it participates in the production by these cells of essential hematopoietic colony-stimulating factors, to be discussed below. Cell-cell adhesion may be mediated by binding of the hematopoietic very late antigen 4 (VLA-4) integrin to stromal fibronectin or vascular cell adhesion molecule 1 (VCAM-1). In addition, cytokine receptors such as KIT can bind to the membrane-bound form of stem cell factor (SCF), and the extracellular matrix proteins secreted by stromal cells may actually provide a binding site for some growth factors or for hematopoietic cells. In addition, chemokines, a family of small molecules, play major roles in stromal function. Specifically, stromal-derived factor 1 (SDF-1) is a potent attractant for hematopoietic cells (both mature leukocytes and progenitor cells that express its CXC chemokine receptor 4 [CXCR4]). Gene disruption studies in mice show that both ligand and receptor are lethal in the embryonic stage, with defects in B lymphopoiesis and myelopoiesis. These relate to a critical role for CXCR4 in bone marrow engraftment in immunodeficient nonobese diabetic/severe combined immunodeficient (NOD/SCID) mice ; SDF-1 has been shown to activate the integrins VLA-4, VLA-5, and lymphocyte function–associated antigen 1 (LFA-1) on CD34+ cells. Last, SDF-1 plays a role in the egress of progenitor cells from bone marrow to blood during stem cell mobilization. Indeed, pharmacologic inhibition of the SDF-1 receptor CXCR4 with the drug plerixafor is employed clinically to enhance mobilization.
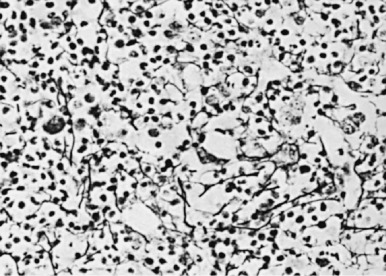
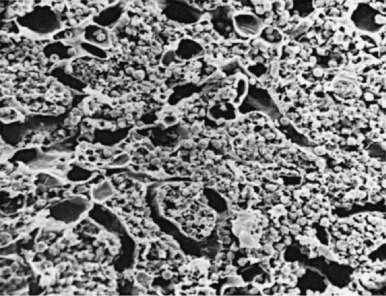
A schema of the marrow circulation is shown in Figure 1-4 . The central and radial arteries ramify in the cortical capillaries, which in turn join the marrow sinusoids and drain into the central sinus. Cells that egress from the marrow sinusoids then join the venous circulation through comitant veins. The inner, or luminal, surface of the vascular sinusoids is lined with endothelial cells, the cytoplasmic extensions of which overlap, or interdigitate, with one another. The escape of developing hematopoietic cells into the sinus for transport to the general circulation occurs through gaps that develop in this endothelial lining and even through endothelial cell cytoplasmic pores.
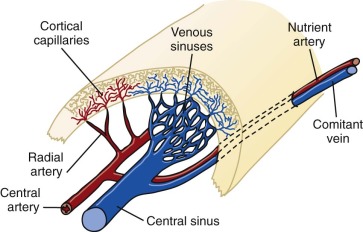
The location of the different hematopoietic cells is not random. Clumps of megakaryocytes are found adjacent to marrow sinuses. They shed platelets, the fragments of their cytoplasm, directly into the lumen. This reduces the requirement for movement of bulky mature megakaryocytes, a mobility characteristic of the granuloid- and erythroid-differentiated precursors as they approach the point at which they egress from the marrow. A schema that illustrates the transfer of hematopoietic cells into the sinus is shown in Figure 1-5 . Disruption of the function of microenvironmental cells inhibits long-term murine marrow cultures. Such disruptions may be responsible for certain cases of aplastic anemia. In recent years significant attention has been paid to the cellular components of the hematopoietic stem cell (HSC) niche, with several reports of the role of osteoblasts as supportive microenvironmental cells that liberate critical cytokines such as SDF-1. However, alternative views argue for a major role of vascular endothelium of the marrow sinusoids in sustaining HSCs (see Fig. 1-5 ). See the review by Krause and Scadden for further details.
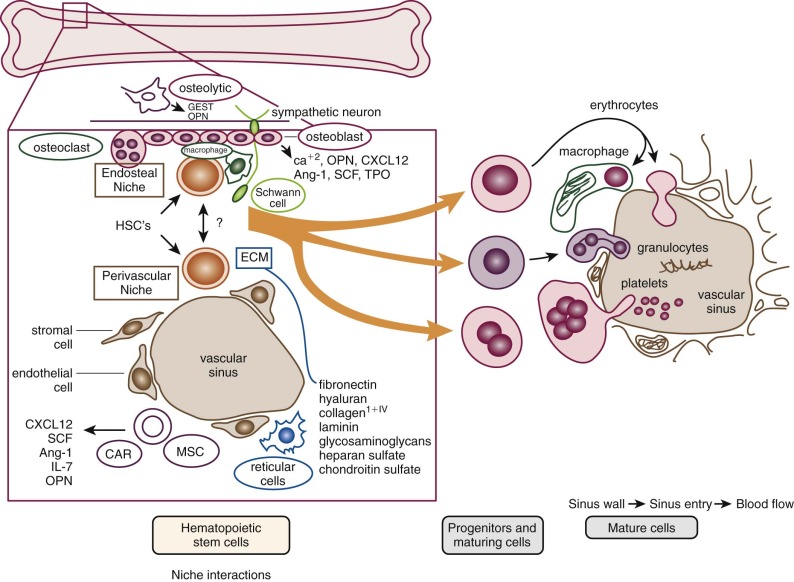
Hematopoietic Cells
Stem Cells
The concept that sustained hematopoiesis derives from pluripotent stem cells was first suggested in 1949 by Jacobson, who showed that mice can be protected from the lethal affects of whole-body irradiation by exteriorization and shielding of the spleen. This protective effect was shown to be cell mediated by the observation that the injection of spleen cells could initiate recovery and reestablish hematopoiesis in irradiated animals. The clonal nature of hematopoiesis and the concept that a single pluripotent stem cell exhibits the capacity to repopulate the entire hematopoietic system was first demonstrated experimentally by Till and McCulloch, who also used the mouse as an experimental system. They found colonies of hematopoietic cells containing erythrocytes, granulocytes, macrophages, and megakaryocytes in the spleens of irradiated recipients within 10 days after transplant. Subsequent experiments using karyotypically marked donor cells confirmed the clonal origin of the differentiated cells in the colony, proving that a single multipotent stem cell had given rise to these differentiated cells. It was also shown that each colony contained a number of stem cells that could again form a colony of differentiated progeny in a second irradiated recipient, demonstrating their self-renewal capacity. This is true only of spleen colonies that are present on days 12 to 14 (colony-forming unit–spleen,[CFU-S 12 ]). Colonies observed on days 7 to 8 after marrow infusion are transient, disappear by day 12, and are neither multipotential nor self-maintaining. Under steady-state conditions no more than 10% of the CFU-S become committed to differentiation during any given 3-hour period. The demonstration of a stem cell that can differentiate to form progenitor cells for erythropoiesis, granulopoiesis, and megakaryopoiesis is consistent with subsequent observations in disease states such as chronic myelogenous leukemia and polycythemia vera, in which a clonal origin of abnormal erythroid, granulocytic, and megakaryocytic precursor cells and lymphocytes can be demonstrated.
The demonstration of a multipotent stem cell in adult bone marrow led to a systematic search for the ontogenic origins of HSCs. Experiments performed by Moore and Metcalf demonstrated the presence of cells capable of repopulating the adult marrow in the yolk sac and the murine fetal liver. Subsequent work has corroborated these observations. It is widely accepted that the first blood cells that circulate in the mammalian embryo arise from the yolk sac. This so-called “primitive” blood lineage consists primarily of nucleated erythrocytes that express embryonic forms of hemoglobin. The classic view of the developmental origins of HSCs holds that the first progenitors of the yolk sac later populate the fetal liver, where they mature to become the “definitive” HSCs of the adult bone marrow, whose erythroid progeny express mature adult forms of beta hemoglobin. However, painstaking dissections of midgestation mouse embryos prior to the onset of blood circulation suggest that the HSCs that can engraft the bone marrow of an irradiated adult mouse first arise within the embryonic trunk. Refinements of the anatomic location of these nascent definitive HSCs show their emergence from a specialized hemogenic endothelium along the base of the developing aorta, where the first pulsations of the heartbeat serve as a trigger to activate an endothelial-to-hematopoietic transition. Debate persists, and some argue that HSCs may arise simultaneously in the yolk sac and intraembryonically from the hemogenic endothelium of the aorta. Complicating the true origins of the first definitive HSCs further, the placenta also appears to be a source of HSCs during development, although it remains possible that the stem cells arrive through the circulation from intraembryonic sources such as the hemogenic endothelium of the aorta. Alternatively, given its rich network of arteries, the placenta may indeed be a de novo producer of blood stem cells. The placenta is quantitatively a large source of HSCs during development.
The most primitive cells with the greatest self-renewal capacity reconstitute long-term hematopoiesis after transfer into irradiated recipient mice. These cells, termed long-term reconstituting hematopoietic stem cells (LTR-HSCs), were shown to be separable from CFU-S 12 in a limiting-dilution assay designed to detect and enumerate “cobblestone areas.” This assay is derived from the original “Dexter” technique for long-term culture of murine marrow in which CFU-S, colony-forming unit–granulocyte-macrophage (CFU-GM), and burst-forming unit–erythroid (BFU-E) flourish for many months on and within an adherent stromal monolayer. The areas of active hematopoiesis have a “cobblestone” appearance. In the limiting-dilution assay, different concentrations of bone marrow cells are plated onto a series of microwells that contain a preestablished stromal monolayer, and at 5 weeks the cobblestone areas that comprise proliferating blast cells within the stromal cell layer are counted. In cell separation experiments, their numbers correlate with a cell fraction that is characterized by low mitochondrial mass per cell (minimal retention of the supravital fluorochrome rhodamine-123); this Rho− cell fraction is enriched for marrow repopulating cells but depleted of CFU-S 12 . Intermediate and rapidly sedimenting cells contained more than 99% CFU-S 12 as well as the cells responsible for short-term reconstitution. In contrast, long-term reconstituting cells (>60 days) came from a slowly sedimenting fraction that contained only 0.25% CFU-S 12 .
The stem cell model of hemopoiesis has parallels in other organ systems. That rapidly self-renewing epithelial tissues such as skin and intestine have stem cells that continually replenish the cells lost by differentiation is well described. However, the demonstration of the existence of neural stem cells has raised the possibility that many organ systems might retain a population of self-renewing stem cells. Muscle satellite cells also appear to fulfill this role. These organ- or tissue-specific stem cells arise during early fetal development from embryonic stem cells of the inner cell mass of the blastocyst that are pluripotent and give rise to all cell types in the body.
Many assays have been proposed as “surrogate” stem cell assays, but until homogeneous populations can be evaluated in both in vitro and in vivo assays it will be impossible to determine the precise cell type measured by these methods. Fauser and Messner and others demonstrated colonies in semisolid media that contain granulocytes, erythrocytes, monocytes, and megakaryocytes (CFU-GEMM) in methylcellulose cultures of human bone marrow. A unique type of in vitro blast cell colony that comprises small numbers of blast cells with higher self-renewal capacity (secondary colonies on replating) than CFU-GEMM has been described. Evidence for the presence of pluripotent HSCs is also derived from the human “Dexter” technique for liquid culture of marrow, in which myeloid progenitors (mostly CFUs-GM) are sustained for about 2 months on and within an adherent stromal monolayer. The progenitors can be detected by replating into methylcellulose with several growth factors at 5 to 8 weeks, thereby demonstrating that unipotent and multipotent cells are generated in this culture system. Eaves and colleagues have adapted this long-term culture technique to a limiting=dilution assay in which long-term culture-initiating cells (LTC-ICs) can be quantitated after culture at different concentrations on a stromal layer for 5 weeks, followed by replating in methylcellulose to score for the number of wells that do not contain colonies. The analogous cobblestone area–forming cell (CAFC) assay has also been adapted to human cells. The high proliferative potential colony-forming cell (HPP-CFC) assay that gives rise to macroscopically visible (>5 mm) in vitro colonies is another method that measures the enormous proliferative capacity of primitive progenitor cells. In an effort to establish a more direct measure of the ability of human stem cells to reconstitute hematopoiesis, Dick and colleagues developed an in vivo assay. In this method human cells are injected into immunodeficient nonobese diabetic/severe combined immunodeficient (NOD/SCID) mice, and 5 to 8 weeks later the animals are sacrificed and tested for the presence of human cells in blood and progenitors in bone marrow. By limiting-dilution assay these SCID reconstituting cells (SRCs) can be quantitated. Because NOD/SCID mice develop thymic lymphomas that prevent long-term studies and have natural killer (NK) cell activity that confers graft resistance, improvements in the model have been achieved by using mice with additional truncated (NOG) or deleted (NSG) interleukin 2 receptor (IL-2R) common gamma chains ; these animals support a -fivefold higher level of CD34+ engraftment. Cell fractionation and gene marking studies provide some evidence that SRCs are more primitive than LTC-ICs. Although SRCs may still be a heterogeneous population of cells that includes long-term repopulating HSCs, this assay provides a better measure of stem cell properties than the LTC-IC assay, especially with regard to the multipotent (i.e., myeloid and lymphoid) and self-renewal properties of HSCs. A tentative relationship of the cells measured in these different assays to the stem cell is shown in Figure 1-6 .
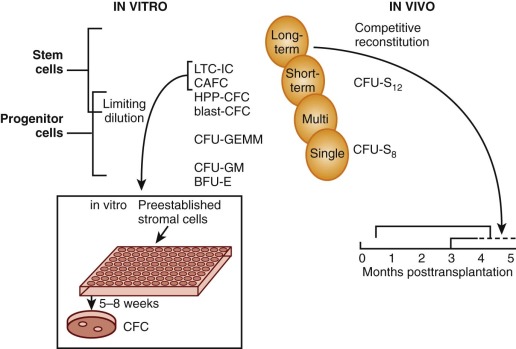
Application of these assays and analysis of HSCs, in general, has been hindered by the low frequency of the cell in the hematopoietic population and the lack of reagents to identify stem cells. However, it is now possible to purify murine stem cells by several methods. Murine HSCs can be highly purified by density gradient centrifugation combined with labeling with antibodies, lectins, or intracellular dyes (alone or in combination) followed by separation using fluorescence-activated cell-sorting (FACS), immunopanning, or immunomagnetic beads. Immunologic reagents that define murine stem cell populations include antibodies to Thy-1 (CD90), Kit, and stem cell antigen (Sca-1). Weissman and coworkers used the FACS in combination with negative expression of T-cell, B-cell, granulocyte, and monocyte lineage-specific markers; low expression of Thy-1; and expression of Sca-1 to isolate HSCs. Transplantation of single Kit + , Thy-1 lo , Lin−, Sca-1 + (KTLS) cells can ensure long-term survival of lethally irradiated syngeneic recipients, and approximately 20% of such cells are LTR-HSCs. Murine HSCs can be even further enriched using cell surface receptors of the signaling lymphocyte activation molecule (SLAM) family. HSCs are CD150+, CD244−, and CD48−, whereas multipotent progenitors lose CD150 expression and begin to express the other family members CD244 and CD48. Purification of CD150+, CD48−, and CD41− cells (CD41 is also expressed on megakaryocyte lineage cells) provides a population of cells 45% of which are LTR-HSCs.
Expression of the CD34 antigen has been used to enrich for human stem cells. Although most colony-forming cells (CFCs) express both the CD34 and CD33 antigens, cells that give rise to CFC in long-term bone marrow cultures (i.e., pre-CFCs) can be separated by their expression of CD34, lack of expression of CD33, and intermediate forward light-scattering properties. A G 0 CD34+ cell population has been isolated by exploiting the resistance of these cells to 5-fluorouracil (5-FU) in the presence of stem cell factor (SCF) and interleukin-3 (IL-3). The G 0 cells are also positive for KIT, interleukin-6 receptor (IL-6R), and IL-1R, and they do not form progenitor-derived colonies upon direct culture in methylcellulose; after 5 weeks in culture on stromal cells, however, they do form primary colonies in methylcellulose 40% of which are replatable. The importance of CD34-positive marrow cells is emphasized by in vivo simian studies. Similar to human bone marrow, the CD34 antigen is expressed by a minority of baboon cells, and infusion of these cells isolated by immunoabsorption chromatography and FACS can reconstitute lymphohematopoiesis in lethally irradiated baboons. The cloning of the murine CD34 complementary DNA (cDNA) has cast some doubt on expression of CD34 by LTR-HSCs, at least in the mouse. A monoclonal antibody raised to murine CD34–glutathione-S-transferase (GST) fusion protein was used to separate purified Sca-1+, Kit+, lin− bone marrow cells into CD34 lo /−, CD34 lo , and CD34+ fractions. Interestingly, long-term multilineage reconstitution was observed after transplantation of the CD34 lo /− cells, whereas the CD34+ fraction gave early but unsustained multilineage reconstitution. These data are supported by experiments demonstrating that a tiny subset of murine bone marrow cells that exclude the Hoechst 33342 dye (called the side population ) contains all the LTR-HSC activity but is CD34−. It is possible that murine and primate LTR-HSCs differ in their expression of CD34; however, the human and primate transplants have not used very highly purified cells, and so it is also possible that CD34 lo /− cells could account for the long-term engraftment. Primate and human studies have also raised the possibility that HSCs do not express CD34. When primitive human lin− cells are separated into CD34+ and CD34− fractions, the capacity to reconstitute hemopoiesis in immunodeficient mice (SRC) is found in both cell fractions. A resolution to this controversy may come from the demonstration that resting murine HSCs are CD34−, whereas HSCs activated with 5-FU or cytokines such as G-CSF express the CD34 antigen. Most interestingly, transplantation of activated CD34+ HSCs showed that these HSCs can lose CD34 expression after return of the recipients to the resting steady state and still retain the capacity to reconstitute secondary recipients, demonstrating that CD34 expression is reversible. The current state of the art for HSC purification in the mouse includes the following marker profile: CD34−, CD150+, CD48−, CD41−, flt3−, and CD49b lo ; whereas in the human system, the current purification would exploit a different marker profile: CD34+, CD38−, CD45RA−, CD90+, and CD49f+, with further enrichment (capacity for single-cell engraftment) in a Rho − fraction of these cells.
Progenitor Cells
The long-term repopulating HSCs of the marrow (LTR-HSCs) slowly self-replicate while occasionally (and stochastically) differentiating into cells that are multipotent but have reduced self-renewal capacity (called myeloid lymphoid progenitors in humans) and then into either common lymphoid progenitors or common myeloid progenitors. These cells can be prospectively isolated according to their expression of unique combinations of cell surface markers ( Fig. 1-7 ). Lymphoid differentiation will not be further considered here. The common myeloid progenitor differentiates into all of the progenitors of the blood cells other than lymphoid cells. These include more committed progenitors of the granulocyte, monocyte (GMPs), and eosinophil lineages or progenitors of the megakaryocyte, erythroid, and basophil lineages (MEPs). These cells can be isolated prospectively, and transplantation into lethally irradiated mice can confer transient but not long-term hematopoiesis.
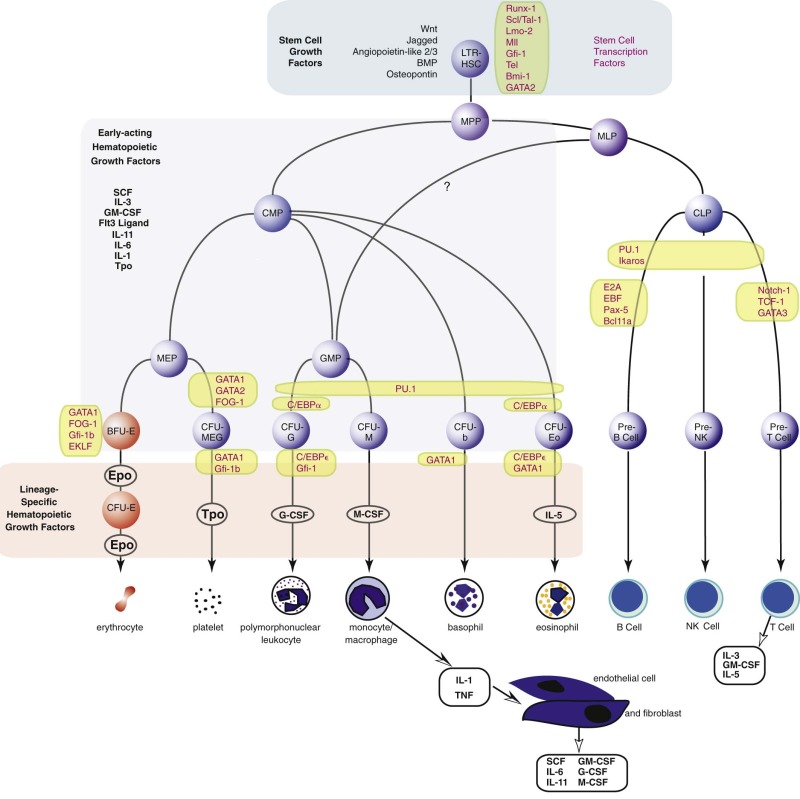
The current view of the mouse hierarchy derived from transplant studies has defined a linear track from LTR-HSCs to short-term reconstituting hematopoietic stem cells (STR-HSCs) (the latter most likely equivalent to the human myeloid lymphoid progenitor), and finally a multipotential progenitor, and then several branch points. There is a lymphocyte-myeloid multipotent progenitor (LMPP) that gives rise to common lymphoid progenitors and GMPs, whereas GMPs can also arise from common myeloid progenitors. There is certainly heterogeneity in these populations, and future fate mapping and sorting techniques may further define the populations.
Erythroid Colony-Forming Cells
The erythroid progenitor compartment is invisible under the light microscope. Erythroid progenitors are committed progenitors of a single lineage derived from the stochastic differentiation of bipotential or multipotential progenitors that are, in turn, derived from a tiny population of multipotential stem cells (see Fig. 1-7 ). In humans, the most primitive single-lineage committed erythroid progenitor is the erythroid burst-forming unit (BFU-E). In response to the combination of erythropoietin and either SCF, IL-3, or GM-CSF in vitro in semisolid (methylcellulose) cultures, BFU-E divide several times while still motile, thereby forming subpopulations of erythroid colony-forming units (CFU-E). Then, each of the latter forms a large colony of proerythroblasts that go on to form more mature erythroblasts and even reticulocytes. The burstlike morphology of the colony is responsible for the name of the progenitor. The entire process requires about 2 weeks in vitro. Bone marrow also contains the more mature CFU-E that, under the influence of erythropoietin, form small colonies of erythroblasts in 7 days.
The murine erythroid progenitor phenotype (pre–CFU-E) can be defined as Sca-1−, Kit+, CD41−, CD150+, and endoglin+. The human BFU-E counterpart is CD34+, CD38+, IL-3Rα−, and CD45RA−.
Granulocyte and Macrophage Colony-Forming Cells
The first colony assays relevant to the study of the production of granulocytes and monocytes in the mouse were described in 1965 by Pluznik and Sachs and in 1966 by Bradley and Metcalf. Analogous assays have been developed in the human system. These groups demonstrated that individual cells derived from mouse spleen or bone marrow could give rise to colonies of up to several thousand differentiated granulocytes and/or macrophages in a soft agar medium. A period of 7 to 8 days was required for full maturation of these colonies (12 to 14 days is required in humans). Appropriate studies were performed to demonstrate the single-cell origin of the colonies. These studies also demonstrated that a single progenitor cell, which was termed the colony-forming unit in culture, or CFU-C, was capable of differentiation into both granulocytes and macrophages, giving rise to the designation CFU-GM. Unit gravity sedimentation and other separation methods have been used to demonstrate that CFU-GM represent a cell population distinguishable from the pluripotent stem cell. Long-term liquid bone marrow cultures have been particularly helpful in defining humoral and cell-cell interactions that induce myeloid differentiation. CFU-GM give rise to the more mature granulocyte and macrophage colony-forming units, CFU-G and CFU-M, respectively. In addition, CFU-GM can be distinguished from the eosinophil progenitor, CFU-Eo, each arising independently from the common myeloid progenitor (CMP). Human CFU-GM are CD34+, CD38+, IL-3Rα lo , and CD45RA−.
Megakaryocyte Colony-Forming Cells
Figure 1-7 provides an accepted if idealized schema of lineage-restricted megakaryocyte progenitor development. Evidence strongly suggests that the initial phase of differentiation into erythrocyte and megakaryocyte commitment involves a single progenitor capable of giving rise to colonies of differentiated cells, all of which express a nuclear transcription factor known as GATA1. Of great interest is the fact that another transcription factor, nuclear factor, erythroid 2 (NF-E2), has been shown to regulate platelet production. Mice rendered NF-E2 deficient have increased numbers of immature megakaryocytes in the marrow but die from bleeding resulting from absence of platelets. The thrombocytopenia is due to a block late in megakaryocyte maturation. Interestingly, these animals do not show an increase in thrombopoietin levels, suggesting that the megakaryocyte mass rather than the platelet count regulates thrombopoietin production.
Precursors and Mature Cells
The erythroid precursor or erythroblast pool represents about one third of the marrow cell population in the normal child above the age of 3 years or in the adult. Proerythroblasts are the earliest recognizable forms. These divide and mature through various stages that involve nuclear condensation and extrusion and hemoglobin accumulation. On average, each erythroblast can form about eight reticulocytes. Measurement of the total marrow proerythroblast content and daily reticulocyte production shows that under normal conditions, replicating proerythroblasts largely maintain the reticulocyte pool being renewed from the progenitor compartment at a rate of about 10% per day.
Erythroid Development
Up to this point we have discussed the nondescript progenitors of erythropoiesis without reference to their physical appearance or to the appearance of their differentiated daughter cells. The best evidence to date suggests that hematopoietic progenitors or stem cells look like lymphoblasts, and studies of peripheral blood have shown that BFU-E reside in the nonadherent “null” lymphocyte population.
The pathway of erythroid precursor differentiation between the development of proerythroblasts and the mature red cell is known as the erythron and includes the functioning differentiated precursor cells observed in bone marrow aspirates and biopsies. The morphology of erythroid precursor maturation is well described in several texts and will not be repeated here. The salient features of the morphologic changes during cell development are related to biochemical and kinetic alterations that were reviewed by Granick and Levere and are shown in Figure 1-8 . The residence times spent in each morphologic compartment are shown at the bottom of the figure, but the average transit time from proerythroblast to emergence of the reticulocyte into the circulation is approximately 5 days. In acute anemia, the transit time may decrease to as little as 1 or 2 days by means of skipped divisions. The red cells that emerge are macrocytic and may bear surface i antigen and other fetal characteristics because the abbreviated time in the marrow compartment does not permit complete conversion of i antigen to I antigen or acquisition of certain other adult characteristics. The cells also contain excessive burdens of the rubbish that normally accumulates during cell assembly, because less time is available for the cleansing action of cell proteases and nucleases. Thus stress erythropoiesis is associated with circulating Pappenheimer bodies (iron granules), basophilic stippling (ribosomes), Heinz bodies (hemoglobin inclusions), and Howell-Jolly bodies (nuclear remnants).
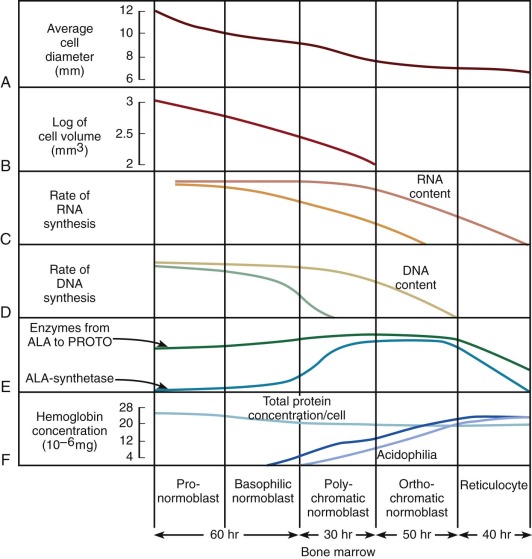
The kinetics of erythropoiesis can be monitored by the use of radioactive iron and surface scanning. The various ferrokinetic patterns in human diseases are shown in Figure 1-9 . The total distribution of erythroid marrow can be determined by scintigraphy using 111 InCl bound to transferrin, as shown in Figure 1-10 . Both 59 Fe kinetics and 111 InCl scintigraphy can be useful in the diagnosis of marrow failure, but these techniques are rarely necessary. The initial uptake of 59 Fe in marrow is found primarily in proerythroblasts and early basophilic erythroblasts.
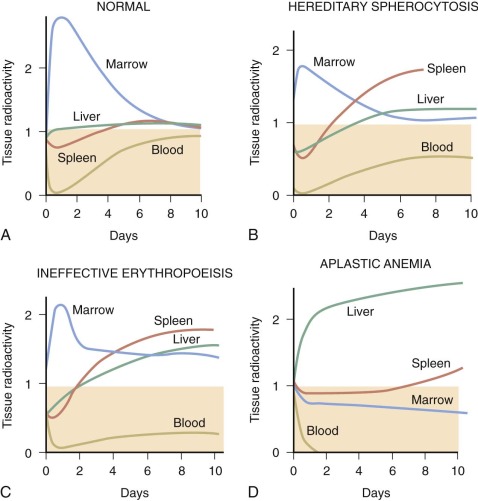
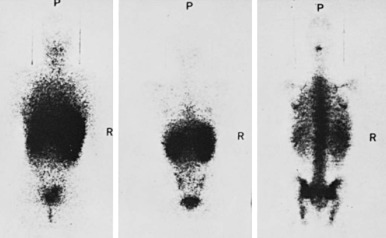
Mouse proerythroblasts; basophilic, polychromatic, and orthochromatic normoblasts; reticulocytes; and mature erythrocytes can be separated on the basis of increasing expression of glycophorin A (Ter119) and loss of expression of CD71. Highly purified populations can be obtained by substituting CD44 for CD71, since there is a thirtyfold loss of expression of CD44 during erythroid maturation compared with a fourfold decrease in CD71 expression.
Neutrophil Production
A model that describes the production and kinetics of neutrophils in humans is shown in Figure 1-11 . It is highly compartmentalized. The relatively tiny peripheral blood pool is divided into two components in equilibrium; the circulatory pool (CGP) and the marginating pool (MGP). These pools provide entrance into the tissues. The level of circulating cells is buffered by an immense marrow reserve of identifiable precursors, some of which are in the mitotic compartment and others in a maturing storage compartment. The transit times within each compartment are relatively long, so that a huge reserve remains available. The responses of these compartments to various diseases are detailed in Chapter 22 . The kinetics of proliferation of recognizable cell precursors have been studied using labeled precursors of DNA. The so-called labeling indices from which measurements of cell cycle times can be made have served as important approaches to the study of the pharmacology and toxicity of chemotherapy (see Chapter 45 ).
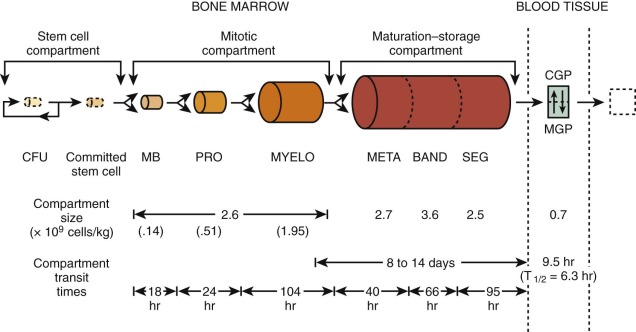
The final stages of granulocyte production and their release from the marrow are also multifaceted. At least four factors may influence granulocyte egress: the organization and localization of the cells in relation to vascular channels; the development of nuclear and cytoplasmic changes that increase cell deformability; factors that cause cell release; and, finally, the regulation of blood flow through vascular channels in the marrow.
Bone Marrow Examination and Megakaryocytopoiesis
Despite the fact that the morphology of megakaryocyte development is fairly well established ( Table 1-1 ), bone marrow examinations can be of limited value in the various platelet disorders. Bone marrow smears and biopsies provide insufficient data about thrombopoiesis because the final stage of platelet production, extrusion of cytoplasm into the sinusoid and shedding of platelets (to be described later), is not appreciated by these techniques; only the relative numbers of megakaryocytes and their size and ploidy can be appreciated by routine morphologic methods. It is not surprising that such information is only loosely correlated with platelet production ( Fig. 1-12 ). Furthermore, sampling errors can be responsible for serious misinterpretations. This is a particular hazard in aspirates of neonatal marrow, in which megakaryocytes may be hard to detect whether platelet production is normal or not. Furthermore, megakaryocytes are not evenly distributed in marrow smears. They are more readily found around the edges of the particles, and they may be mistaken for broken cells by the untrained observer. Megakaryocyte nuclei are often found lying free in marrow smears, where they may be erroneously scored as tumor cells. Biopsy sections provide more accurate assessments of megakaryocyte number and distribution than smears, though the latter are usually sufficient (except in neonates) if examined carefully. Biopsies should not be attempted in neonates merely to define megakaryocytes; clinical judgment is a safer tool in these patients.
Stage | Nuclear Morphology | Cytoplasmic Staining (Wright-Giemsa) | Approximate Size Range | Demarcation Membranes | Granules | Suggested Name |
---|---|---|---|---|---|---|
I | Compact (lobed) | Basophilic | 6-24 µm | Present by electron microscopy | Few present by electron microscopy | Megakaryoblast |
II | Horseshoe | Pink center | 14-30 µm | Proliferating to center of cell | Starting to increase | Promegakaryocyte |
III | Multilobed | Increasingly more pink than blue | 15-56 µm | Extensive but asymmetric | Great numbers | Granular megakaryocyte |
IV | Compact but highly lobulated | Wholly eosinophilic | 20-50 µm | Evenly distributed | Organized into “platelet field” | Mature megakaryocyte |
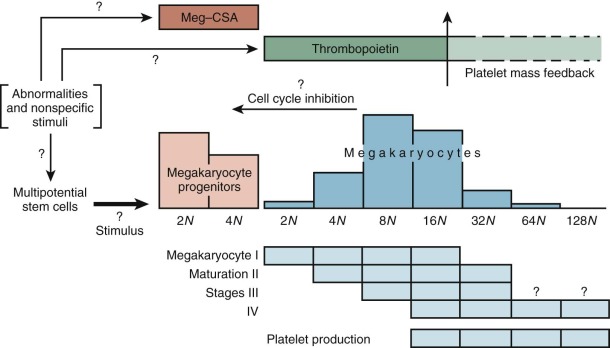
Examinations of routine marrow smears and biopsies, though instructive, are limited by their two-dimensional views and the thickness of the sections. Megakaryocytes have a peculiar predilection to lie next to the endothelial cell lining of the fronds of developing marrow cells, perhaps because thrombopoietin is produced by these cells. In general, megakaryocytes are too large to squeeze through the sinusoidal meshwork, so they merely push their cytoplasms through the fenestrations. These cytoplasmic structures, called proplatelets, serve as microtubule conduits for platelet packaging and release at sinusoids. The protruding cytoplasms form demarcation lines and then shatter into platelets, which are swept into the blood, regulated in part by fibrinogen interacting with platelet integrin αIIbβ3. The megakaryocyte nuclei rarely make the transfenestration journey into the sinusoids and thence the blood. If they do, they may be mistakenly interpreted by the unwary microscopist as tumor cells in the blood. Intact or partial megakaryocytes are regularly observed in the blood of patients with marrow-invasive diseases such as certain leukemias, metastatic cancers, granulomatous disorders, and fibrosis.
The Hematopoietic Growth Factors
Since the pioneering work in the early 1960s by Metcalf and Sachs and their coworkers, it has been recognized that normal and leukemic blood progenitor cells can be propagated in culture in the presence of soluble protein growth factors. These factors were originally termed colony-stimulating factors, or CSFs, based on their ability to support the formation of colonies of blood cells by bone marrow cells plated in semisolid medium. During the 1970s and 1980s, it was recognized that there exist multiple types of CSFs based on the different types of blood cells found in the colonies that grew in the presence of the different factors, leading to the hypothesis that the growth and differentiation of different lineages of blood cells are controlled, at least in part, by exposure of progenitor cells to CSFs having different lineage specificities. With the molecular cloning of the genes for many of these factors and their receptors during the 1980s and 1990s it became possible to study in detail the structure, function, and biology of the recombinant CSFs as well as the molecular biology of their respective genes. This analysis, along with similar work on the regulation of cells in the immune system, led to the realization that there exist a large number of interacting regulatory molecules now generally known as cytokines or lymphohematopoietic cytokines that together serve to control the hematopoietic and immune systems and to integrate the responses of these systems with those of many other physiologic systems. These control molecules serve to regulate the growth, development, differentiation, activation, and trafficking of cells within the hematopoietic and immune systems. With the elucidation of the sequence of most of the human genome in the final years of the 1990s, even more cytokine and growth factor genes have been discovered, providing new insight into how all these systems have evolved with common themes but also providing further challenges to cell biologists in their attempts to understand the functions and interactions of all of the different molecules.
The cytokine gene families that contain at least one member that functions within the hematopoietic or immune system include the lymphohematopoietic cytokines (including many interleukins and CSFs ); the receptor tyrosine kinase ligand CSF-1, the stem cell factor (SCF) (also known as steel factor ), and the ligand for the Flk2/Flt3 receptor (FLT-3) ; the IL-1 gene family ; the chemokines ; the tumor necrosis factors ; the interferons ; the IL-10 gene family ; the transforming growth factor β (TGF-β) ; and the IL-17 gene family. We will briefly describe these families and the molecules among the family members selected as hematopoietic growth factors (HGFs) for further discussion.
The Lymphohematopoietic Cytokines
For our purposes, the lymphohematopoietic cytokines are defined as the protein ligands for the members of the hematopoietin receptor gene family, also known as the cytokine receptor class I gene family . This gene family is characterized by an extracellular domain with pairs of conserved cysteine residues and the distinctive amino acid sequence element Trp-Ser-Xaa-Trp-Ser (WSXWS). These receptors all signal at least partly through the JAK-STAT pathway. This rather large family of hematopoietins now includes many interleukins (IL-2, IL-3, IL-4, IL-5, IL-6, IL-7, IL-9, IL-11, IL-12, IL-13, IL-15, IL-21, and IL-23), granulocye-macrophage colony-stimulating factor (GM-CSF), granulocyte colony-stimulating factor (G-CSF), erythropoietin (EPO), thrombopoietin (Tpo), thymic stromal thymopoietin (TSLP), leukemia inhibitory factor (LIF), oncostatin M (OSM), cardiotropin 1 (CT-1), and ciliary neurotrophic factor (CNTF). The cytokine receptors on the cell surface signal as multimers, either homomeric or heteromeric, and the family can be further divided into subfamilies based on the molecular composition of their receptor complexes. We will focus on cytokines that have major actions on hematopoietic cells. Those with homodimeric receptors include EPO, Tpo, and G-CSF. A second subgroup comprising GM-CSF, IL-3, and IL-5 in the human system have receptor complexes that share a common subunit known as the beta common chain that forms heterodimers with unique cytokine alpha subunits to give selectivity for the respective cytokines. A third group includes IL-6 and IL-11 (as well as LIF, OSM, CNTF, and CT-1, not considered further); all share a common receptor-signaling chain known as gp130, with unique chains that provide ligand specificity.
The Receptor Tyrosine Kinase Ligands
Three HGFs, macrophage CSF (M-CSF, also known as CSF-1 ), stem cell factor (SCF), and the ligand for FLT3 (FLT3L) have been identified that signal through related receptors, which themselves have tyrosine kinase activity. The receptors for these HGFs are all evolutionarily related, as are the ligands themselves, and each plays an important role in controlling blood cell production.
The Interleukin 1 Gene Family
The IL-1 gene family has grown substantially and now includes at least many IL-1s (IL-1α through IL-1ξ), the IL-1 receptor antagonist (IL-1RA), and IL-18. IL-1α, IL-1β, and IL-18 all have potent ability to induce cytokine production (IL-18 particularly interferon gamma (IFN-γ) in combination with IL-12 ), but the two IL-1s have also been shown to synergize with other HGFs in support of early blood cell proliferation and, therefore, will be considered further here. The functions of the other novel members of the IL-1 gene family are still unknown.
The Chemokines
The chemokines represent a large family of generally small proteins (usually 8 to 12 kDa) readily identifiable by sequence homology, including twin characteristic cysteine residues near the amino termini that separate the family into CC chemokines if the cysteines are adjacent or CX chemokines if they are separated by one residue. Currently, there are more than 50 members of the gene family that have demonstrated important roles in cell trafficking in many systems. The receptor gene family for this cytokine family comprises about 20 members of the G-protein–coupled receptor (GPCR) family. However, their biologic function is not limited to chemotaxis, because several have been shown to effect gene transcription, apoptosis, and cell proliferation. IL-8 is a prominent CX chemokine that regulates neutrophil trafficking and therefore plays a role in inflammatory responses. However, two chemokines, SDF-1 and macrophage inflammatory protein 1α (MIP-1α) have been reported to inhibit the cycling of very early stem cells and therefore may be important molecules in halting cycling stem cells and returning them to their normal, quiescent state. SDF-1 is clearly very interesting and important in the hematopoietic system as a chemotactic factor for very primitive stem cells, providing possible mechanisms for the homing of cells both as hematopoiesis moves from the fetal liver to the bone marrow and during transplantation of cells in adults.
The Tumor Necrosis Factors
The TNF gene family now includes TNFα, lymphotoxin α and β, CD27Ligand, CD30Ligand, CD40Ligand, FasLigand, TRAIL, and Trance. Many of these molecules are involved in regulating activation and death of various cells, including T lymphocytes and erythroid progenitors. TNFα itself has many biologic activities, particularly in inflammation. However, it is mentioned here because of its potent and important role in promotion of dendritic cell differentiation and activation and its capacity to depress hematopoiesis.
The Interferons
The type I interferons (13 species of IFN-α, IFN-β, and IFN-ω) are closely linked on human chromosome 9. The type II interferon gamma (IFN-γ) demonstrates little if any sequence homology with the type I interferons, but the receptors for all of these cytokines are members of cytokine receptor class 2, which is related to but distinct from cytokine receptor class I (HGF receptors) because class 2 receptors lack the distinctive WSXWS sequence. In addition to their antiviral activities, both type I and type II interferons have multiple other regulatory activities with many cell types, including T cells. In the human system, IFN-γ plays a prominent role in polarizing T helper cells toward the type 1 (Th1) phenotype. However, none of these molecules appear to have prominent roles in directly controlling blood cell production and will not be considered further here.
The Transforming Growth Factor Beta Gene Family
The large TGF-β gene family includes TGF-β1, TGF-β2, and TGF-β3; the bone morphogenetic proteins (BMPs) 2 through 16; the growth and differentiation factor (GDF) genes 1 to 10; activin; and inhibin. Like those for many of the other cytokines, the receptors for the TGF-β family members are multimers comprised of two different members of the serine/threonine kinase receptor gene family. Receptors of different ligand specificities are generated by forming these gene products in different combinations. TGF-β1 itself is a very important regulator of cell cycling in many cell systems, including the hematopoietic system, and will be considered here further as a negative regulator of hematopoiesis. Mutations in its receptor cause Loeys-Dietz syndrome. The BMPs and GDFs have been implicated in many developmental systems. Interestingly, BMP-4 has been shown to be essential in Xenopus species for the embryonic development of HSCs, and it seems at least possible that this molecule might also play a role in mammals in blood cell development during embryogenesis if not in adult hematopoiesis, an area worth following. However, for our purposes and the sake of simplicity, we will only include TGF-β1 as an HGF.
Actions of Hematopoietic Growth Factors
Predominantly Lineage Specific HGFs: G-CSF, M-CSF, IL-5, TPO, and EPO
Early on, it was recognized that different colony-stimulating factors could selectively support the growth of specific types of hematopoietic colonies. Thus when human bone marrow cells are cultured in semisolid medium in the presence of G-CSF, colonies emerge 7 to 8 days later that consist largely of mature neutrophilic granulocytes and their precursors. This finding led to the model that G-CSF, to a large degree, interacts with relatively late hematopoietic progenitors that have already committed to the neutrophil lineage (CFU-G) and thus G-CSF supports their growth and final maturation into functional neutrophils. Similar analysis has revealed that the other major hematopoietic cell lineages have analogous, lineage-specific, late-acting factors and that these molecules frequently serve as important if not primary regulators of the respective pathways. Thus M-CSF supports monocyte/macrophage colony growth and is important in supporting the growth and maturation of monocyte progenitors (CFUs-M) ; IL-5 supports eosinophilic granulocyte colony formation and therefore supports the growth and maturation of eosinophil progenitors (CFUs-Eo) as well as activating eosinophils ; EPO is necessary for the growth and maturation of both earlier (BFU-E) and later (CFU-E) progenitors of the erythroid lineage ; and TPO directly supports the growth and maturation of megakaryocyte progenitors (CFUs-meg) and the subsequent production of functional platelets.
Although the regulation of the respective blood cell pathways by the lineage-specific HGFs is likely to be their major function, in no case is this lineage specificity absolutely maintained. G-CSF has been found to influence the migration and proliferation of endothelial cells, cells that express high-affinity receptors for this cytokine. IL-5 serves as a growth factor for activated B cells, particularly in the mouse, and affects the type of immunoglobulin secreted by mature B cells. Epo and TPO have been noted to interact with megakaryocyte and erythroid progenitors, respectively. M-CSF appears to be important in trophoblast development. Finally, populations of early HSCs have been found to express receptors for many cytokines; typically, these cells do not respond to single factors but require combinations of factors to trigger them into cycle. “Lineage-specific” factors that have been reported to act in various combinations to trigger cycling of early “stem” cells include G-CSF, M-CSF, and TPO, demonstrating that the molecules are not strictly “lineage specific” even within the hematopoietic system. Mice deficient in either the Tpo receptor ( Mpl ) or G-CSF are deficient in levels of all progenitor cells, consistent with the idea that these factors indeed are involved in expansion of early lineage cells. However, when administered in vivo each of these molecules largely influences the growth and development of the expected lineage, so that the designation of lineage specificity seems warranted.
Multilineage Hematopoietic Growth Factors: IL-3 and GM-CSF
Initial analysis of human bone marrow cell cultures grown in the presence of GM-CSF revealed that a variety of different colony types develop over a period of 10 to 14 days. Mature blood cells that could be readily identified included neutrophils, monocytes/macrophages, and eosinophils; this finding led to the designation of the molecule as a “granulocyte-macrophage” colony-stimulating factor, or GM-CSF. In comparison with G-CSF, it was found that it took longer to produce colonies with identifiable neutrophils but the ultimate variety of cell types was greater. This led to a model in which GM-CSF acts on progenitors committed to produce either neutrophils or monocytes (CFU-GM), a precursor to the G-CSF–responsive CFU-G and the M-CSF–responsive CFU-M. These later progenitors apparently retain responsiveness to GM-CSF as well because mature monocytes and neutrophils can be observed in cultures supported by GM-CSF alone. That this model is not strictly correct was shown when recombinant GM-CSF was introduced into human bone marrow cultures in the presence of EPO and it was found that this combination of factors was very effective in supporting the development of erythroid colonies (murine GM-CSF is somewhat less effective in this regard). Thus despite its name, GM-CSF generally interacts with intermediate multilineage progenitors that yield neutrophils, eosinophils, monocytes, erythroid cells, and megakaryocytes (CFU-GEMM). At the time, these activities were similar to those ascribed to IL-3 in the murine system. When human IL-3 was identified, it proved to have abilities to support multilineage colony formation similar to those of human GM-CSF, indicating that it interacts with slightly different but strongly overlapping subsets of progenitors. In comparison with GM-CSF, IL-3 is somewhat more effective in supporting multilineage, erythroid, and megakaryocyte colony formation and GM-CSF is slightly more effective with granulocyte and monocyte/macrophage colony formation. In serum-free conditions, the ability of IL-3 to support final neutrophil and monocyte maturation is significantly depressed, indicating that the later-acting factors, G-CSF or GM-CSF in the case of neutrophils or M-CSF or GM-CSF in the case of monocytes, are necessary for final end cell production.
In addition to acting slightly earlier than GM-CSF, IL-3 is clearly distinguished in its activity by its ability to support the growth and maturation of mast cells and basophils. In the mouse, this was one of the first recognized activities of IL-3, and when IL-3 was first administered to primates, basophilia was one of the most prominent findings. Thus IL-3 appears to be capable of supporting the growth and development of basophil and mast cell progenitors. In the human system, both IL-3 and GM-CSF can support the development and differentiation of dendritic cells of either myeloid or lymphoid origin, especially in combination with SCF, FLT3L, and TNF.
Other Interleukins
IL-4 in mice and humans has been reported to support multilineage colony formation, including colonies that contain cells from the erythroid, megakaryocytic, neutrophilic, and monocytic lineages. IL-4 in the mouse supports mast cell growth and therefore shares many activities with IL-3. However, IL-4 plays very important roles in the development and maturation of T cells and B cells, particularly in the polarization of cytokine production by helper T cells toward the type 2 (Th2) response. Therefore, on balance IL-4 is likely to be more important in controlling immune cell development and function and will not be discussed further here. IL-9 in both the murine and human systems has been shown to enhance erythroid colony formation in the presence of Epo and appears to play a role in T-cell and B-cell development as well. More recently, IL-9 has been shown to have some effects on the growth and activation of mast cells and may play some role in the pathology of asthma.
Early-Acting Hematopoietic Growth Factors: Stem Cell Factor and Flt3 Ligand
Stem cell factor (SCF) and FLT3 ligand (FLT3L), both receptor tyrosine kinase ligands, interact with a variety of hematopoietic progenitor cells, perhaps most importantly with very early stem cell populations. SCF also plays an important role in melanocyte growth and development, which is reflected in the coat color effects of mutations in SCF or its receptor, KIT. Genetic analysis of mice clearly showed that mice defective in either SCF (Sl mice ) or in Kit (W mice ) have serious hematopoietic (and many other) defects, including macrocytic anemia, mast cell deficiencies, and deficiencies in the stem cell compartment (reviewed in reference 152). These early studies had already indicated the critical importance of SCF in the survival and development of stem cells. Mutations in the human KIT gene lead to a similar phenotype in melanocyte development in humans known as the piebald mutation; however, these individuals do not have any hematologic problems, probably because severe mutations in this locus are likely to be lethal. In vitro, the activities of SCF are generally most evident when combined with other HGFs; the proliferative activity of SCF with hematopoietic cells in culture as a single factor is minimal. In fact, culture of murine bone marrow cells in SCF alone ultimately yields largely mast cells. However, SCF acts synergistically to enhance the activities of most of the other HGFs in culture and is particularly effective when combined with HGFs such as IL-3, IL-1, or IL-11 at promoting the expansion of “blastlike cells” that retain considerable potential for yielding multilineage colonies in secondary culture. These colonies, when replated under conditions that support B-lymphocyte development or when transplanted into animals also yield B and T lymphocytes, indicating that SCF-responsive cells include primitive stem cells with both lymphoid and myeloid potential. SCF has also been implicated in combination with IL-2 or IL-7 in early stages of T-cell development in the thymus, with IL-7 in pre–B-cell growth, and with IL-7 in enhancing NK cell responsiveness to IL-2. However, the fact that none of these lineages are dramatically affected in W or Sl mice indicates that SCF-independent mechanisms can compensate in these systems.
SCF, M-CSF, and FLT3LG are all expressed as both membrane-bound and soluble forms. In the case of SCF, expression of membrane-bound forms of the molecule in the marrow microenvironment provides a nice model for how this growth factor might act locally. Indeed, cell lines that express membrane-associated SCF exclusively are much more effective in supporting long-term hematopoiesis in vitro than cell lines that produce soluble forms of the molecule exclusively. This interaction of membrane-associated SCF with KIT provides one mechanism for the adherence of hematopoietic cells to stroma; binding of human megakaryocytes to fibroblasts can be blocked by antibodies to KIT. Finally, if membrane-associated forms of SCF are present in which the cytoplasmic domain is essentially missing, the result is male but not female sterility, suggesting that the cytoplasmic domain may have an as yet undetermined important biologic function.
As shown by the early genetic studies and confirmed through analysis of the recombinant protein, SCF is not specific for the hematopoietic system. It is an important growth factor for melanocytes and primordial germ cells, and it appears to play a role in development of the nervous system, perhaps as a neuronal guidance factor, although it has been difficult to demonstrate neurologic defects in W or Sl mice other than the fact that the mice are severely constipated due to SCF dependence of intestinal Cajal cells.
The FLT3 receptor tyrosine kinase was originally identified as a novel receptor present in HSCs; with human marrow, the expression is largely limited to the CD34+ cell population. FLT3L alone yields low numbers of CFU-GM colonies from human bone marrow but acts synergistically with other cytokines, including IL-3, GM-CSF, EPO, and SCF, to yield enhanced colony formation, both in terms of size and numbers of colonies. The synergy observed between FLT3L and the other HGFs is comparable to that observed with SCF in similar systems, with the exception that FLT3L has little effect on BFU-E. Multifactor combinations with SCF have been used for expansion of colony-forming cells in long-term cultures; FLT3L has effects comparable to those of SCF when combined with IL-1, IL-3, IL-6, and EPO in 4-week cultures. Like SCF, FLT3L in combination with other cytokines such as GM-CSF supports dendritic cell development from CD34+ bone marrow cells. In contrast to SCF, FLT3L does not support the growth and development of mast cells. Despite this overlap in bioactivities, mice in which the Flt3 receptor tyrosine kinase has been disrupted appear to have normal hematopoiesis, with the only detectable defects observed within the B-lymphocyte lineage. However, mice in which both the Kit and Flt3 receptor tyrosine kinase genes have been disrupted display more severe hematologic complications than mice with mutation of only one of these genes, suggesting that the two pathways can to some degree compensate for one another. The importance of FLT3L in B-cell growth has also been shown with cultures of primitive B-cell progenitors (CD43+, B220 low ) in combination with either IL-7 or SCF. Altogether, these findings argue for an important role for FLT3L in hematopoiesis.
Other Stem Cell Factors: Wnt, Jagged, BMP, and Angiopoietin-like Growth Factors
Several new growth factors have been shown to regulate HSC production during embryogenesis as well as adulthood. The wnt family of factors regulates stem cell homeostasis. Overexpression of wnt 3A led to a threefold expansion of HSCs. This is the first time that stem cell number was actually increased in a competitive repopulation assay. In separate experiments, overexpression of β-catenin led to an expansion of HSCs, and sorting by activated β-catenin in HSCs based on a wnt reporter line that turned cells green when wnt was activated demonstrated HSC activity. Further studies in chronic myeloid leukemia (CML) demonstrated that wnt activation was present in leukemia. These studies demonstrated that wnt activity could drive HSC production; however, other experiments have demonstrated that the knockout of β-catenin within the HSCs is not required for stem cell homeostasis. Two papers demonstrated that a constitutively activated β-catenin in HSCs leads to aplastic anemia. The activation of the wnt pathway may not be necessary for stem cell homeostasis, but it may be sufficient to drive the HSCs to produce more self-renewal cell divisions.
The wnt pathway clearly interfaces with HSC production. It is possible that pulses of wnt will have different activity than a constitutively active wnt signal. In addition, redundancy within the marrow space among other signaling pathways, including the notch and BMP pathways, has left the stem cell compartment with significant chances of survival. Wnt acts through canonical and noncanonical pathways. In the former case this leads to activation of β-catenin to the nucleus through the complex involving adenomatous polyposis coli (APC) and β-catenin. In the latter, there is stem cell activation by the Rho GTPases. Wnt activation acts at multiple places during hematopoiesis, at the stem cell level, at the pre-B-cell level, and at multiple places in T-cell development. Wnts bind to canonical frizzled receptors and activate a signaling pathway. An antagonist wnt signal, wnt 5a, suppresses B-cell proliferation and may act to suppress stem cell homeostasis. Other wnts have been found to regulate hematopoiesis, including wnt 2b and wnt 10b for human bone marrow CD34+ cells.
The notch pathway has been shown to regulate HSC production based on retroviral transduction of hematopoietic cells. This expands the population of HSCs significantly. Notch works through a ligand receptor interaction in which deltas and jaggeds are presented on the stem cell niche. In general, jagged2 is thought to be the predominant notch ligand in the marrow and is presented by the osteoblast. Modulation of jagged2 leads to an expansion of HSC production. The notch pathway has been put into a genetic pathway with runx1, based on the zebrafish mutant mindbomb. Mindbomb mutants have no notch signaling due to a defect in an E3 ubiquitin ligase that promotes ligand recycling. This mutant lacks HSCs during development, and overexpression of runx1 rescues hematopoiesis. A pulse of notch signaling has been shown to expand HSCs in the zebrafish system. Furthermore, the runx1 rescue of notch 1 mutant was demonstrated in the mouse system and it was also shown that GATA2 was a target of the notch pathway. Modulation of wnt and notch demonstrates synergy of wnt 3a and activation of the notch pathway. It is possible to activate wnt pathways using the chemical called BIO drug, which is an inhibitor of glycogen synthase kinase 3beta GSK-3β. This modulation of the wnt and notch pathways represents a significant role in stem cell homeostasis.
The bone morphogenetic protein family has been demonstrated to induce HSC production in the Xenopus and zebrafish systems. This leads to an expansion of the erythroid blood islands during early development. Interestingly, a dominant-negative version of BMP activated later in development leads to enhanced erythropoiesis, demonstrating two independent effects of BMP regulation of HSC differentiation and proliferation. Furthermore, BMP has recently been shown to expand HSC production in the aorta-gonads-mesonephros region. BMP was shown to expand or maintain HSC production in human embryonic stem cells and functions as a key regulator of stress-induced erythropoiesis. BMP also regulates the stem cell niche. It is unclear how BMP and the notch and wnt pathways interact.
Angiopoietin-related proteins are potent activators of HSC production. Thus angiopoietin-like 2 and angiopoietin-like 3 were shown to lead to a twentyfold or thirtyfold expansion of long-term HSCs when they were cultured for 10 days in the presence of the ligands. This was demonstrated by a limiting-dilution competitive repopulation assay. Thus angiopoietin-like genes must also participate in the stem cell niche. Another growth factor glycoprotein called osteopontin was also involved in stem cell homeostasis; it negatively regulates the stem cell pools. A number of factors, including TGF-β1, have a negative regulation as well. Ligands produced by the stem cells themselves may also regulate stem cell homeostasis.
Synergistic Factors: IL-1, IL-6, and IL-11
Early in the 1980s, activities were identified that had the ability to enhance hematopoietic colony formation supported by other HGFs, particularly with early progenitor cells. One activity, designated hematopoietin 1, was subsequently purified and discovered to be IL-1. In this fashion, IL-1 was recognized as having little ability on its own to stimulate hematopoietic colony formation but was able to act in synergy with other HGFs, notably IL-3, in increasing both the frequency of colony formation and the numbers of cells per colony. Subsequent to the discovery of the synergistic activity of IL-1, numerous other cytokines have emerged with similar activity, including IL-6, IL-11, leukemia inhibitory factor (LIF), and IL-12. Of these, IL-6, IL-11, and LIF all signal through a common signal-transducing molecule, the gp130 component of the IL-6 receptor. Because these molecules are likely to behave similarly in most systems and because IL-6 and IL-11 have been the most thoroughly studied, we will limit our discussion to these two members of this family. IL-12, which signals through a distinct but perhaps similar pathway to gp130, has interesting effects in combinations with other cytokines but appears to be more important in regulating the development and activities of T and NK cells and will therefore not be discussed further here.
The effects of IL-1 on hematopoiesis have been highly complicated by the fact that this cytokine is a potent inducer of secondary cytokine production, frequently by accessory cells in the culture. The induction of other growth factors, notably IL-6, G-CSF, GM-CSF, and IL-11, is likely to contribute to the activity of IL-1 as a synergistic factor in hematopoietic colony formation. Nevertheless, combinations of IL-1 with other factors in cultures of highly purified hematopoietic cells typically yield synergistic effects, suggesting that at least some of the effects are direct. However, even with highly purified hematopoietic progenitor cell populations, the effects of IL-1 can be indirect. For example, Rodrigues and colleagues have reported that IL-1 can prevent apoptotic death of CD34+ lin− human bone marrow cells but the effect is largely abrogated by antibodies to GM-CSF, suggesting that some progenitor cells in the population can produce their own GM-CSF. Thus the survival effect of IL-1 in some systems may also be indirectly mediated by induction of GM-CSF expression in CD34+ lin− bone marrow cells themselves.
IL-6 is an extremely pleiotropic cytokine with important effects on the growth and differentiation of T and B cells, on the induction of the hepatic acute-phase response, and on the enhancement of the proliferation of hematopoietic progenitor cells. IL-6 signaling, mediated by two members of the hematopoietin receptor gene family, IL-6R and gp130, was the first example of signaling through a commonly shared receptor subunit, gp130, which now is known to be involved in the signaling of LIF, IL-11, oncostatin M (OSM), ciliary neurotrophic factor (CNTF), and cardiotrophin-1 (CT-1). Because most cells express gp130, the expression of other receptor components such as IL-6R or IL-11R generally determines whether or not a cell will respond to one of these family members; cells that express receptors for multiple members of this cytokine subgroup generally exhibit identical or nearly identical responses to each member whose receptor component is expressed by the cell.
IL-6 has little if any ability to support colony formation on its own but can enhance colony formation supported by other HGFs, particularly IL-3 and SCF. This effect was most prominent using bone marrow cells from mice isolated for 2 days following treatment with 5-FU, a drug that enriches for primitive progenitors by selectively killing later, actively cycling cells in the bone marrow. Bone marrow cells treated in this fashion generally yield significant numbers of colonies only when plated in the presence of multiple HGFs. In this system, IL-6 was found to enhance colony formation supported by IL-3 or SCF. These early cells are typically quiescent in the G 0 phase of the growth cycle, and combined effects of cytokines, such as IL-3 and IL-6, are required to push them into active cycling. Similar effects have been observed with cultures of purified early human hematopoietic progenitor cells.
As noted above, the IL-11 receptor complex employs the IL-6 gp130 signal-transducing system. Thus IL-11 and IL-6 appear to have somewhat overlapping biologic activities. In general, IL-6 has proved to have more effects on T and B cells than IL-11 and similar effects in the hepatic acute-phase response and on osteoclast formation, but IL-11 is somewhat more potent in megakaryocytopoiesis. Like IL-6, the effects of IL-11 in hematopoietic cultures are largely observed only in combination with other factors. IL-11 in combination with SCF or FLT3L has proved to be very effective in supporting the growth of primitive hematopoietic progenitors. Soon after its discovery, IL-11 was shown to have important effects on the growth and development of megakaryocyte progenitors. Again, in this system, IL-11 had little effect on colony formation on its own but was found to act in synergy with IL-3, SCF, or, more recently, TPO in supporting CFU-meg colony formation. Its clinical use in thrombocytopenic states has been disappointing.
Negative Regulator of Hematopoiesis: Transforming Growth Factor Beta
A variety of cytokines have been reported to reversibly inhibit cycling of early hematopoietic cells, including the chemokines SDF-1, MIP-1α, and TGF-β, perhaps the most potent of all. Very interestingly, when individual early cells were plated in wells, either antibody to TGF-β1 or an antisense oligonucleotide to the mRNA for this factor could trigger the release of the cells from quiescence in the presence of appropriate combinations of early-acting cytokines, which by themselves alone failed to trigger the cycling of the cells.
Molecular Biology of Hematopoietic Growth Factors
Genomic Analysis of the Cytokine and Cytokine Receptor Genes
With analysis of the human genome, several common themes and relationships have emerged from the different types of cytokine/growth factor and receptor genes. First, as we have already noted, most of the cytokines and their receptors exist as members of distinct, evolutionarily related gene families; examples of a single cytokine or growth factor gene with no relatives are rare. Similarly, the receptors also fall into gene families. Thus a relatively small number of primordial growth factor/cytokine genes were duplicated many times, forming families of genes that evolved into serving regulatory functions for many different systems. Interestingly, in most cases, each of the individual cytokines of a particular gene family use members of a single receptor gene family. For example, all of the chemokines use G-coupled protein receptors as their signaling/recognition molecules, and all of the members of the TGF-β gene family use different combinations of the serine/threonine kinase receptors as their recognition and signaling molecules. All of these receptor-ligand pairs must have evolved in concert as they were selected to control different cellular processes.
Despite the obvious gene duplications that have occurred in all of these gene families, there is no absolute rule as to how the genes are arranged today. In some cases, the related genes are still closely linked in tandem in the genome, as is the case for the IL-3 and GM-CSF genes on chromosome 5 at 5q31.1 ( Fig. 1-13 ). In this case, another duo of related genes, IL-4 and IL-13, are very closely linked to one another but are slightly more separated from the IL-3/GM-CSF cluster.
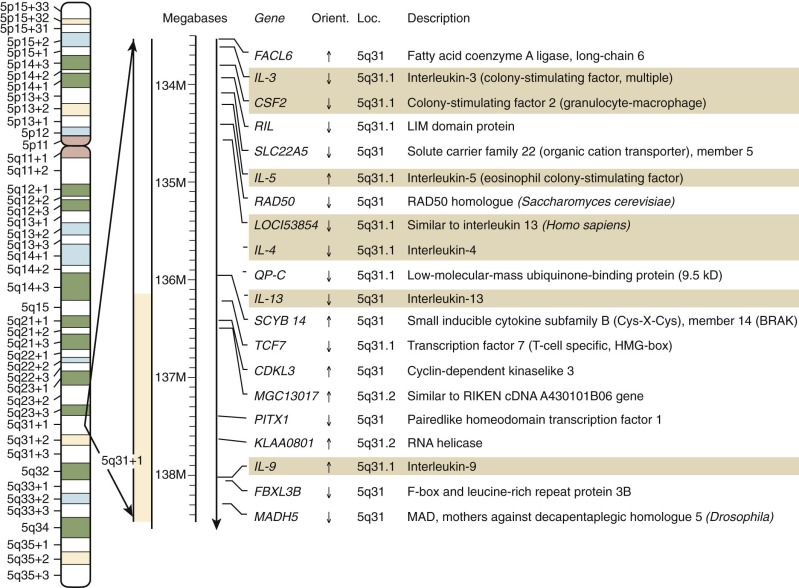
Structures of the Hematopoietic Growth Factor Proteins
The availability of the cDNAs for the individual cytokines allowed for the rapid determination of their primary amino acid sequence, for their expression in quantities large enough for direct x-ray determination of their crystal structures, and, more broadly, for predicting structures based on those of proteins related even distantly by sequence. One of the clear results of this analysis of the structures of the different cytokines was the realization that, despite generally low conservation of amino acid sequence, many of the lymphohematopoietic cytokines are distantly related in evolution. Most of the lymphohematopoietic cytokines are believed to assume a tightly packed, antiparallel, four—α-helix–bundled structure with either short or long helical bundles. Lymphohematopoietins having the short helical bundle structure include IL-2, IL-3, IL-4, IL-5, IL-7, IL-9, IL-13, IL-15, and GM-CSF; cytokines having the long helical structure include the gp130 signaling proteins IL-6, IL-11, LIF, and OSM; IL-12p35; and the homodimeric receptor signaling proteins EPO, TPO, and G-CSF.
Sequence and structural information suggest that the cytokines with the long helical bundle structure are all distantly related in evolution. Members of the IL-6 subfamily, including IL-6, IL-11, and G-CSF, show rather modest sequence similarity but display common gene organization and certain structural features that suggest evolutionary relatedness. Sequence alignments of IL-6 and G-CSF show conservation of cysteine residues. Interestingly, a cytokine identified in the chicken, myelomonocytic growth factor (MGF), displays sequence similarities with G-CSF and IL-6, which suggests that all three are derived from a common ancestral gene. Erythropoietin shows some structural features similar to those of growth hormone and to G-CSF but at a very low level. In contrast, the amino-terminal half of TPO shows strong sequence similarity with EPO, wherease the carboxy-terminal half is unrelated to any known protein. The four-helical-bundle structure of EPO is clearly related to the amino-terminal, helically structured portion of TPO, whereas the additional domain of TPO, which is heavily glycosylated, may serve to increase the serum half-life of the molecule. Both of these family members are believed to act as monomers.
The ligands for the receptor tyrosine kinase gene family members, SCF, FLT3L, and M-CSF, share many structural similarities. All three contain transmembrane domains and are initially synthesized as homodimeric integral membrane proteins. Although the membrane-bound forms are functional, dimeric soluble forms that also display biologic activity are released from the cell by proteolysis. The extracellular domains display low but significant sequence similarity, including conservation of the positions of the cysteine residues involved in disulfide bridge formation. Modeling of these extracellular domains indicates that they, too, are likely to form four α-helix–bundled structures tethered to the membrane through variable spacer domains, with dimerization through a cysteine located in the spacer domain.
Hematopoietic Growth Factor Gene Disruptions
Hematopoietin Receptor Ligands
G-CSF Gene. The combination of technology for disrupting genes in cultured mammalian cells has led to methodology for studying gene function in vivo (mutants related to HGFs are summarized in Table 1-2 ). Using this approach, Lieschke and associates were able to generate mice with both copies of the G-CSF gene disrupted. These animals develop normally but as adults display a 70% to 80% reduction in levels of circulating neutrophils, a 50% reduction in the numbers of progenitors in all lineages, and impaired resistance to challenge to infections with Listeria monocytogenes . These findings, which are in agreement with earlier observations by Hammond and colleagues in dogs that had developed cross-reacting antibodies to canine G-CSF, demonstrate the central role for G-CSF in controlling neutrophil levels. However, the decline in all populations of progenitors is consistent with a role for G-CSF prior to commitment to the neutrophil lineage, perhaps at the early stem cell stage.
A. Human Mutations | ||||
Gene | Mutation | Phenotype | Reference | |
IL2Rγc | Deletion | X-linked severe combined immunodeficiency (SCID) | ||
JAK3 | Deletion | SCID | ||
GCSFR | C-terminal deletion | Kostmann disease developing leukemia | ||
EPOR | C-terminal deletion | benign erythrocytosis | ||
B. Nonhuman Mutations | ||||
Species | Gene | Name | Phenotype | Reference |
Mouse | Kit | White-spotting | Macrocytic anemia, mast cell deficiency, lack of pigmentation, sterile | |
Sl | Steel | Macrocytic anemia, mast cell deficiency, lack of pigmentation, sterile | ||
Csf1 | Microphthalmia | Osteopetrosis due to decreased osteoclast function | ||
Hcp | Motheaten | Immunodeficiency, autoimmune disease, increased sensitivity to EPO | ||
Drosophila | JAK homologue | Hopscotch | TumL allele, gain-of-function mutation that causes leukemia | |
C. Targeted Disruption in Murine Embryonic Stem Cells | ||||
Gene | Phenotype | Reference | ||
Il-6 | Decreased CFU-S and stem cell function | |||
Gmcsf | Hematopoiesis normal; progressive accumulation of pulmonary surfactant | |||
Gcsf | Neutrophils 25% normal; impaired response to Listeria monocytogenes infection | |||
Epo | Embryonic lethal; failure of definitive erythropoiesis | |||
Epor | Embryonic lethal; failure of definitive erythropoiesis | |||
Tpor (Mpl) | Platelets 15% to 20% normal | |||
IL-3/-5/GMRβc | Similar to Gmcsf −/− mice; also low eosinophils and impaired eosinophil response to N. brasiliensis | |||
Vav | Not required for hematopoiesis |
Erythropoietin and Thrombopoietin. The TPO and EPO genes have clearly evolved from a common ancestor; at some point the extra protein-coding region found in the final coding exon of the TPO gene was either removed (in the case of the EPO gene) or added (in the case of the TPO gene) following the early duplication event.
Mice with deletion of either the Tpo or Mpl gene have been described. Although these animals develop normally and do not display signficant abnormalities in bleeding times, they do have only 15% to 20% of the normal levels of circulating platelets. This result implies that TPO is very important for maintaining circulating levels of platelets but that other cytokines might have some capacity to generate platelets at low levels. TPO has also been shown to have effects on stem cell homeostasis. In the case of Epo , gene disruption results in embryonic lethality due to failure of fetal liver erythropoiesis.
IL-6–gp130 Complex Ligands: IL-6 and IL-11. Animals that have been engineered to lack the IL-6 gene develop normally but display significant deficiencies in various immune and inflammatory responses. The hepatic acute-phase response in these animals is severely compromised after tissue damage but only moderately in response to lipopolysaccharide (LPS) The effects of disruption of the IL-6 gene on normal hematopoiesis are relatively minor; a slight decrease in peripheral blood leukocyte counts, a 10% reduction in bone marrow cellularity, a 50% reduction in CFU-S d12 , and a fourfold to fivefold reduction of pre–CFU-S.
GM-CSFR Complex Ligands: IL-3, GM-CSF, and IL-5. The genes for each of these proteins comprise approximately 3000 base pairs divided among four exons (GM-CSF and IL-5 ) or five exons (IL-3 ) with somewhat similar structures. All of these genes have been localized to the long arm of chromosome 5 at 5q31.1, a region commonly disrupted or deleted in patients with various malignant myeloid neoplasms. This region also contains the genes for other important cytokines, including IL-4, IL-9, IL-12p40, and IL-13. Detailed mapping of the region has demonstrated that several of these genes are very closely linked: the GM-CSF and IL-3 genes are tandemly arrayed within 9 kb of one another; the IL-4 and IL-13 genes are separated by only 12.5 kb. This clustering of molecules with similar structures and functions, including the sharing of receptor components in the case of the GM-CSF , IL-3 , and IL-5 genes, provides further strong support for the evolutionary relatedness of their respective genes.
Mice with homozygous disruption of the GM-CSF gene develop normally and display neither numerical defects in the levels of circulating granulocytes or monocytes nor defects in the levels of CFU-GM progenitors in marrow or spleen. These animals exhibit alveolar proteinosis with surfactant accumulation in the lungs based on defective aveolar macrophage function. GM-CSF plays an irreplaceable role in the function and development of certain macrophage populations, but any function played by this cytokine in controlling basal or stimulated production of granulocytes or monocytes can be replaced by other factors.
To evaluate the role of the entire IL-3/GM-CSF/IL-5 complex, Nishinakamura and coworkers engineered a mouse lacking the beta-c receptor component shared by all three cytokines as well as a disruption in the IL-3 gene. Mice lacking beta-c show a pulmonary alveolar proteinosis-like disease and reduced numbers of peripheral eosinophils, which are explained by the lack of GM-CSF and IL-5 functions, respectively. Combination with the IL-3 gene disruption showed no further abnormalities in hematopoiesis, demonstrating that the entire IL-3/GM-CSF/IL-5 axis is dispensable for hematopoiesis both in normal and stressed mice.
Receptor Tyrosine Kinase Ligands: M-CSF, Stem Cell Factor, and FLT3 Ligand. The M-CSF , SCF , and FLT3 genes are evolutionarily related. The gene for M-CSF proved to be defective in the spontaneous mutant osteopetrosis ( op/op ). Female op/op mice are infertile, confirming the role of M-CSF in the biology of the pregnant uterus. They also have a severe deficiency in the ability to develop osteoclasts derived from the monocytic lineage, resulting in the development of osteopetrosis and the failure to develop teeth. The animals do develop some macrophage populations, indicating that M-CSF is important in many but not all macrophage-related lineages. The observation that some of the defects in the op/op mouse are corrected as the animals mature has led to speculation that alternative splicing of the mutant M-CSF transcript can lead to production of some functional M-CSF. The OP9 stromal cells are often used in the expansion of stem cells, showing that M-CSF suppresses stem cell growth.
Naturally occurring mutations in the SCF locus ( Sl ) or in the receptor for SCF, Kit ( W ), result in profound effects on many stages of hematopoiesis, most notably in the stem cell, erythroid, and mast cell compartments. These studies have clearly demonstrated the central role of SCF in controlling hematopoietic cell function. Interestingly, disruption of the Flt3 receptor gene has relatively minor effects on hematopoiesis, the most notable being somewhat depressed levels of B-lymphocyte precursors. However, mice with disruption of both the Kit and Flt3 receptor tyrosine kinase genes display more severe hematologic complications than mice with a mutation of only one of these genes, suggesting that the two factors do, at some level, complement one another in vivo.
Hematopoietic Growth Factor Genes: Regulation of Expression
Expression of Hematopoietic Growth Factors by Monocytes/Macrophages and Dendritic Cells: G-CSF, M-CSF, GM-CSF, IL-6, and SCF
Monocytes/macrophages and related cells, including dendritic cells, Kupfer cells in the liver, and Langerhans cells in the skin, are key cells that serve many important functions in regulating the immune system. Among the functions performed by these cells is the production of many cytokines, including TNF, IL-1, G-CSF, M-CSF, GM-CSF, IL-6, and IL-12, in response to various stimuli, including LPS, IL-1, IL-3, GM-CSF, and M-CSF. Other cytokines, including IL-4, IL-10, and IL-11, downregulate monocyte production of many of these cytokines. Several of the cytokines, including IL-18, various members of the IL-17 gene family, and IL-23, are playing important roles in controlling cytokine production by monocytes and macrophages.
All of these interactions are key components of the interactions between T cells and monocytes in determining the nature and direction of the immune response. Different regulators have been found to induce different subsets of these cytokines. For example, LPS has been shown to induce expression of IL-1, IL-6, TNF, IL-1 receptor antagonist, GM-CSF, and G-CSF. In contrast, IL-3 and GM-CSF failed to induce the expression of either GM-CSF or G-CSF but were found to induce M-CSF expression. M-CSF has been shown to induce peritoneal macrophages to activate expression of GM-CSF and IL-6 at the transcriptional level, but additional signals are necessary to induce the release of the functional cytokine proteins from the cells. Murine bone marrow–derived macrophages have been reported to constitutively express the messenger RNA (mRNA) for M-CSF and SCF, and the level of expression can be enhanced by treating the cultures with pokeweed mitogen.
The different HGF genes in monocytes can be upregulated by a variety of different mechanisms. In the case of IL-6, LPS and Mycobacterium tuberculosis activate gene expression at the transcriptional level through activation of the transcription factors NF–IL-6 and NF-κB. In contrast, activation of IL-1 and IL-6 expression by Salmonella typhimurium porins is largely mediated by mRNA stabilization. Cytokine activation of monocyte IL-6 expression also occurs by various mechanisms, often involving interactions between NF-κB and NF–IL-6. IL-2, IL-3, and GM-CSF have all been shown to induce monocyte expression of IL-6, and this expression is inhibited posttranscriptionally by treatment with IL-4. In the case of G-CSF, expression can be activated by treatment of monocytes with either IL-1 or LPS (IFN-γ potentiates the LPS response ); IL-4 given simultaneously can block this induction, an effect that is not mediated by mRNA stability but rather is mediated at the transcriptional level. These interactions between monocytes and T cells and the cytokines they produce, although complicated, are clearly important in determining the direction and extent of the resulting immune responses and are likely to play a key role in controlling the cytokine network.
Dendritic cells, which have been characterized as potent antigen-presenting cells, are also an important source of HGFs and other cytokines. With the availability of cytokines such as FLT3L, GM-CSF, IL-3, and IL-4, it has become much easier to generate dendritic cells from both myeloid and lymphoid sources in significant numbers for further studies. Originally believed to be largely of myeloid cell origin, it is now recognized that some are likely derived from myeloid cells and others have their origins in the lymphoid lineages. Interestingly, different types of dendritic cells, matured under different conditions, can serve to polarize cytokine production by maturing T-helper cells and therefore are likely to play key roles in determining the outcomes of immune responses.
Different populations of fibroblasts, endothelial cells, and epithelial cells can be stimulated to produce cytokines by treatment with LPS, phorbol esters, or other cytokines, including IL-1, TNF, and platelet-derived growth factor (PDGF). In many tissues, this is likely to be an integral part of the host response to infection: Cytokine production at the site of infection should result in local activation of host defense effector cells and the systemic recruitment of more effector cells until the infection is cleared. In bone marrow, thymus, and spleen, these cell populations are likely to be critical components of the local microenvironment, which is involved in the normal proliferation, development, and differentiation of cells of the hematopoietic and lymphopoietic systems. The control of production of the various HGFs in the steady state is still poorly understood.
Fibroblasts and endothelial cells are important sources of many of the HGFs, including G-CSF, M-CSF, GM-CSF, IL-6, IL-11, SCF, and FLT3L. This expression is regulated both transcriptionally and posttranscriptionally by exposure to LPS, phorbol esters, or cytokines such as IL-1, TNF, and IFN-γ. SCF expression has been reported to be constitutive in human stromal bone marrow cultures, in endothelial cells and in bone marrow– derived fibroblasts, and is not responsive to IL-1.
Although it is not clear that stromal fibroblasts from bone marrow are any more or less capable of cytokine production than fibroblasts from other tissues, because of their proximity to stem and progenitor cells they have long been studied for their ability to express HGFs. Many of these cells constitutively express the tyrosine kinase receptor ligands M-CSF and SCF.
Following myeloablation, circulating levels of many HGFs increase dramatically. In some cases this might result from mechanisms that sense low levels of the various blood cells. However, Hachiya and colleagues have found that in culture, TNF and IL-1 synergize with irradiation to upregulate the expression of GM-CSF both through mRNA stabilization and activation of gene transcription. These observations raise the possibility that the myeloablative agents themselves may directly induce or enhance HGF production in vivo following various cancer therapy regimens.
Endothelial cells are another important source of HGFs. Human endothelial cells produce G-CSF, GM-CSF, and M-CSF in response to inflammatory cytokines such as IL-1 and TNF. In contrast to G-CSF and GM-CSF, SCF mRNA is expressed constitutively by human umbilical vein endothelial cells (HUVECs). The levels of SCF mRNA are further increased in response to inflammatory mediators, including IL-1 and LPS. This induction results predominantly from mRNA stabilization by approximately threefold. Increased expression of GM-CSF mRNA in HUVECs is also at least in part mediated by mRNA stabilization. Possibly the induction of SCF mRNA in endothelial cells contributes to the elevated plasma levels of this factor in patients with sepsis or inflammatory diseases.
Regulation of EPO Expression by Hypoxia
Erythropoietin has long been recognized as the physiologic regulator of red cell production. It is produced in the kidney and in the fetal liver in response to hypoxia or exposure to cobalt (II) chloride; the mechanism of the switch of production from predominantly fetal liver to predominantly kidney in adults is largely unknown. Beck and Semenza showed that the Epo gene contains a hypoxia response element in the 3′ flanking sequence.
Nuclear factors present in hypoxic but not normoxic cells were identified as a heterodimeric basic helix-loop-helix transcription factor complex designated hypoxia-inducible factor–1 (HIF-1), comprising inducible HIF-1α and constitutively expressed HIF-1β. HIF-1 is induced in a variety of cell types in response to hypoxia or cobalt, indicating that while it is important in the activation of EPO expression, it is also important in the activation of many other hypoxia-inducible genes. HIF-1 has been shown to bind to an enhancer sequence located approximately 130 bp downstream from the poly-A addition signal of the Epo gene. This enhancer segment has been shown to render other promoter-reporter gene constructs responsive to hypoxia, with typical inductions in the range of 4-fold to 15-fold, significantly less than the 50-fold to 100-fold induction observed with the chromosomal Epo gene in Hep3B cells. In addition to HIF-1 and its role on the 3′ Epo gene enhancer, other studies have identified a 53-bp sequence from the Epo promoter that confers oxygen sensitivity (sixfold to tenfold inducibility) to a luciferase reporter gene. Combining the enhancer and promoter sequences results in cooperative (fiftyfold) inducibility of transcription in response to hypoxia, approaching that observed in vivo.
HIF-1α protein levels are regulated by binding to the von Hippel–Lindau (VHL) tumor suppressor followed by ubiquitin-mediated proteosomal degradation ( Fig. 1-14 ). Under normoxic conditions, a prolyl hydroxylase uses molecular O 2 , iron (Fe II), and α-ketoglutarate to hydroxylate Pro402 and Pro564 in human HIF-1α, which leads to VHL binding and proteosome-mediated proteolysis. A second hydroxylation of Asn 803 by an asparaginyl hydroxylase (similarly dependent on O 2 , Fe II, and α-ketoglutarate) blocks the C-terminal interaction of HIF-1α with the transcriptional coactivators p300/CBP. Under hypoxic conditions both hydroxylases are inhibited, leading to escape from ubiquitin-mediated proteolysis and an increase in HIF-1α protein, as well as recruitment of the p300/CBP and transcriptional activation.
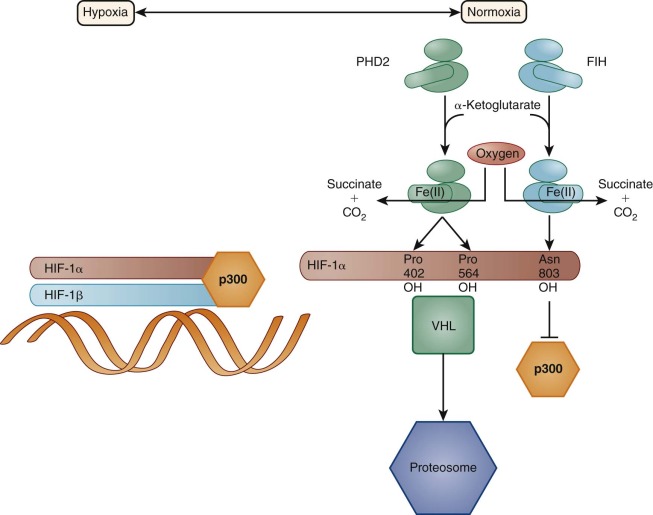
Regulation of Thrombopoietin Gene Expression
The cloning and expression of the gene for thrombopoietin has provided new insight into the regulation of levels of platelets. Gene disruption studies of Mpl , the receptor for TPO in mice, have shown that in the absence of the function of this pathway, mice have only 15% of the normal levels of circulating platelets. Thus while redundancy among the growth factors, perhaps including SCF, IL-3, IL-6, and IL-11, can partially compensate for dysfunctional Mpl signaling, TPO, like EPO in the erythrocyte lineage, appears to be the major regulator of circulating platelet levels. However, in contrast to the control of EPO production, Tpo gene expression does not seem to be transcriptionally regulated or significantly influenced by platelet levels. It may be regulated by the megakaryocyte/platelet mass. In adult mice, the major sources of Tpo mRNA are the liver and kidney; in both organs, the gene is constitutively expressed and is not significantly upregulated during thrombocytopenia. However, circulating levels of TPO increase rapidly during thrombocytopenia and decline as platelet counts return to normal. The observation that platelets themselves can remove TPO from thrombocytopenic plasma in vitro has led to the model that TPO is constantly produced and released into circulation but, in normal circumstances, is rapidly removed by the circulating platelets and possibly megakaryocytes as well. During thrombocytopenia, the platelet shortage fails to remove TPO as fast as it is made, resulting in elevated levels and stimulation of platelet production. This mechanism is similar to one proposed some years ago by Stanley and associates for the regulation of circulating M-CSF levels directly through consumption by the monocytes themselves.
Hematopoietic Growth Factor Receptors
Types of Hematopoietic Growth Factor Receptors
Analysis of the actions of the HGFs on purified murine or human stem and progenitor cells (see below) has shown that there is considerable overlap in the action of the HGFs. Some insight into the apparent overlap in biologic activities of many cytokines has come from analysis of their structural homologies and from the cloning of many of their receptors. These receptors are all type 1 membrane glycoproteins with extracellular N-termini and single transmembrane domains, and they fall into two major classes. Receptors (Rs) such as KIT, FMS (the M-CSFR), and FLT3 are characterized by a cytoplasmic tyrosine kinase domain (RTKs), whereas most of the other receptors lack cytoplasmic tyrosine kinase activity and can be divided into four subclasses ( Table 1-3 ). Most of the HGFRs (class 1) fall into a superfamily with structural features based on two linked fibronectin (FBN) type III domains ( Fig. 1-15 ). Analogous FBN domains are found in the IFN (class 2) receptors, and the class 1 and 2 structures probably evolved from a common primitive adhesion molecule. Although the TNF family of receptors (class 3) are characterized by an extracellular fourfold repeat of approximately 40 amino acids that contains 6 conserved cysteine residues (Cys repeat), the IL-1Rs (class 4) feature extracellular immunoglobulin-like repeats. Although the discussion below is focused mainly on the HGFRs, the RTKs activate many of the same signaling cascades.
Type | Receptor | Cytokine |
---|---|---|
Tyrosine Kinase (Ig repeats) | KIT, M-CSFR (FMS) | Four-α-helix bundle |
Non–Tyrosine Kinase | ||
Class 1: HGFRs (FBN III domains) | Four-α-helix bundle | |
Shared βc | IL-3R, GM-CSFR, IL-5R | |
Shared gp130 | IL-6R, LIFR, IL-11R, IL-12R, OSMR | |
Shared γc | IL-2R, IL-4R, IL-7R, IL-9R, IL-15R | |
Single chain | G-CSFR, EpoR, TpoR (MPL) | |
Class 2 (FBN III domains) | IFNα/βR, IFNγR | |
Class 3 (Cys repeats) | TNFR I, TNFR II, FAS, CD40 | β-jellyroll wedge |
Class 4 (Ig repeats) | IL-1R I and II | β-trefoil fold |
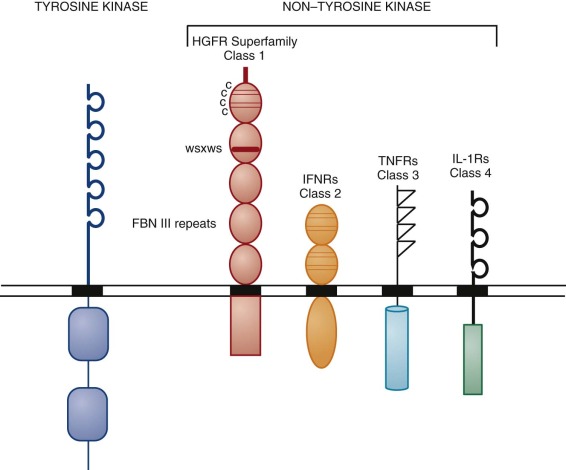
Structure and Binding Properties of Receptors
Prototypes for the class 1 structure are the (nonhematopoietic) prolactin and growth hormone receptors. These polypeptides share a number of features ( Fig. 1-16 ). The major homology lies in the extracellular domain, which is characterized by four conserved cysteine residues in the N-terminal FBN III repeat and a Trp-Ser-X-Trp-Ser (WSXWS) motif in the linked C-terminal FBN III repeat that forms a hydrophobic hinge between the two domains. In addition, the IL-6 family of receptors has members that each have an N-terminal immunoglobulin-like domain as well as extra FBN III repeats. The cytoplasmic regions of the receptor family show much less homology, although membrane proximal domains rich in prolines (box 1, within 20 amino acids of the membrane) and acidic residues (box 2), separated by a positionally conserved tryptophan, have been defined. Mutations within the box1/2 domains inactivate the mitogenic function of the receptors that have been examined, and mutations of the conserved tryptophan inactivate some receptors but not others. An additional interesting feature of many of the HGFRs is that receptor subunits are shared. GM-CSF, IL-3, and IL-5 all have receptors with unique alpha chains that bind their respective ligands with low affinity. They share a common beta chain (βc) that converts ligand binding from low to high affinity in each case and is thought to be critical for signal transduction. A similar arrangement is evident with the IL-6, LIF, OSM, IL-11, and CNTF receptors, all of which share a common beta chain, namely gp130. The finding of shared subunits explains to a certain extent why the actions of GM-CSF and IL-3 overlap on many cells, and why IL-6, LIF, and IL-11 all share pleiotropic actions on HSCs and hepatic cells. The gamma chain of IL-2R is now known to be shared by the IL-4, IL-7, IL-9, IL-15, and IL-21 receptors. Other members of the HGFR superfamily that act on lineage-restricted cells, such as EPOR, G-CSFR, and thrombopoietin (TPO)R, appear not to require an additional subunit for ligand binding or signal transduction.
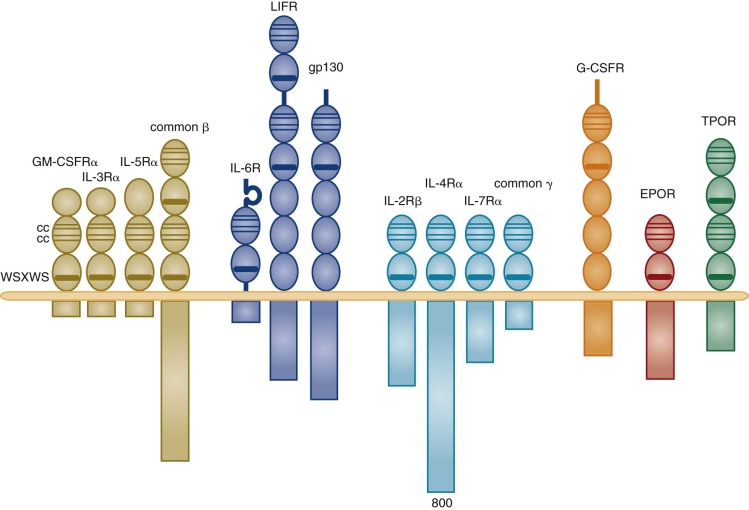
The number of IL-3, GM-CSF, G-CSF, and EPO receptors per cell is strikingly low (≈1000 sites per cell), whereas those for M-CSF are about one log higher. In all cases affinity of a receptor for its ligand is high, with dissociation constants usually in the picomolar range. Stimulation of target cells can occur at concentrations of factor that are orders of magnitude lower than the equilibrium constant at which 50% of receptors are occupied, and therefore it is apparent that low receptor occupancy is sufficient to produce biologic effects.
Receptor Function
Lineage-Specific Factors and Induction of Differentiation
Do lineage-specific receptors direct differentiation, or do intracellular proteins specific for lineage-restricted cells have that function? With respect to receptor-driven events, a proximal cytoplasmic domain of the G-CSFR is essential for proliferation, whereas a more distal domain is important for induction of acute-phase plasma protein expression when the receptor is transfected into hepatoma cell lines, or granulocyte-specific proteins when it is introduced into murine IL-3–dependent factor-dependent cell (FDC-P1) cells. Support for a role for the G-CSFR in granulocyte differentiation comes from sequence analysis of the receptor in two patients with severe congenital neutropenia (Kostmann disease) who went on to develop AML. Two different point mutations in the G-CSFR gene resulted in truncations of the C-terminal cytoplasmic region of the receptor and coexpression of both mutant and wild-type genes. The mutation was present in the neutropenic phase in one of the patients, suggesting that the mutation was not acquired along with the leukemia. Functional analysis by transfection of mutant or wild-type genes into murine 32D.C10 cells showed that the mutation acted as a “dominant negative” and prevented differentiation in response to G-CSF. Other evidence for an inductive role of receptors comes from murine long-term bone marrow cultures infected with a retroviral Fms vector that yielded a pre–B line with an immunoglobulin heavy-chain gene rearrangement. This line grew in IL-7 or M-CSF; interestingly, the switch to M-CSF led to macrophage maturation, suggesting that signals from this receptor can determine differentiation in these bipotent cells. Last, transduction and stable expression of the EPOR in IL-3–dependent Ba/F3 cells results in cells that produce globin mRNA upon EPO stimulation but not IL-3 stimulation ; and a chimeric receptor that comprises the extracellular domain of GM-Rα and the cytoplasmic domain of the EPOR can induce increased glycophorin A expression in Ba/F3 cells, in contrast to the GM-Rα/βc control. Although these experiments suggest that elements of the cytoplasmic domains of these receptors can direct both proliferation and differentiation, experiments with cell lines must be interpreted with caution. Definitive proof that the EPOR is not specifically required for erythroid differentiation comes from retroviral expression of the prolactin receptor in wild-type and Epor −/− progenitors; these cells differentiate efficiently in prolactin, indicating that there is no unique instructive role for the EPO in erythropoiesis. However, the prolactin receptor activates similar signaling pathways to the EPOR such as STAT5, and therefore could perhaps readily substitute for EPOR function. In contrast, GM-CSF cannot rescue lymphopoiesis in GM-CSFR transgenic mice crossed with IL-7–deficient animals; furthermore, common lymphoid progenitors that express ectopic GM-CSFR differentiate into myelomonocytic cells, indicating plasticity in common lymphoid progenitors and an instructive role for GM-CSFR in this context.
Taken together, it is reasonable to conclude that the major role of HGFRs is the survival, the amplification, and, particularly in the case of the lineage-specific receptors, the completion of an intrinsic differentiation program of committed progenitor cells. There is, however, overlap of expression, as with EPOR expression on megakaryocyte progenitors.
Signal Transduction
Hematopoietic Growth Factor–Induced Tyrosine Phosphorylation. Several signaling proteins and pathways are common to many different receptor types. A paradigm common to receptors both with and without endogenous tyrosine kinase activity is that ligand binding induces homodimerization or heterodimerization of receptor subunits, and this is followed rapidly by transient tyrosine phosphorylation of the cytoplasmic domain of the receptor itself, of cytoplasmic tyrosine kinases, and of other cytoplasmic proteins involved in generating different signaling cascades ( Fig. 1-17 ). In the case of the receptors with intrinsic tyrosine kinases, ligand-induced activation of their catalytic function leads to transphosphorylation or autophosphorylation of dimerized receptor subunits and activation of three major signaling cascades: RAS/MAP kinase; phosphoinositol (PI)-3′ kinase/protein kinase B (PKB, also known as AKT ); and phospholipase C (PLC)/inositol trisphosphate (IP 3 )/diacylglycerol (DAG)/protein kinase C (PKC). Receptors that lack a tyrosine kinase domain must recruit cytoplasmic tyrosine kinases. For the class 1 cytokine receptors the family of Janus kinases (JAKs) fulfills this role, but other nonreceptor kinases have been identified and may also be important. Tyrosine phosphorylation within the receptor cytoplasmic domain creates docking sites for substrates characterized by the presence of src homology 2 (SH2) domains. These SH2 domains recognize phosphotyrosine in the context of specific short sequences of amino acids. This leads to activation of the three signaling cascades mentioned above (RAS, PI -3′ kinase, and PLC) as well as the activation by JAK of a critical family of s ignal t ransducers and a ctivators of t ranscription, the STAT proteins.
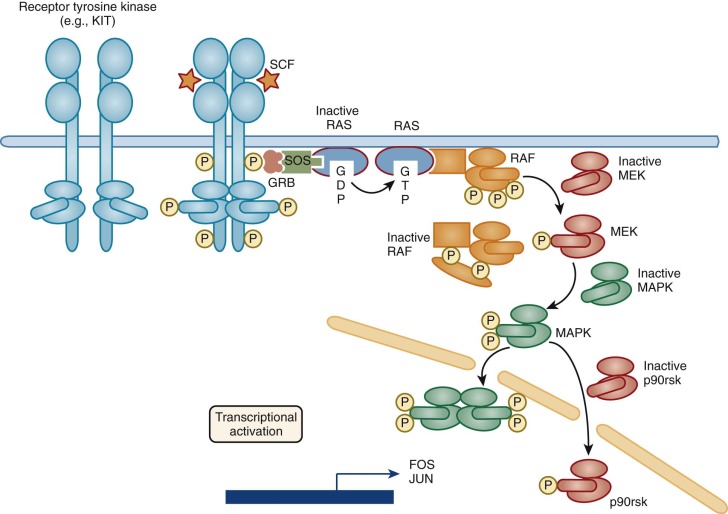
There are four known members of the JAK family, JAK1, JAK2, JAK3, and TYK2. All are 130- to 134-kD related proteins that have an amino-terminal receptor-binding domain, a carboxy-terminal kinase domain, and a middle domain that regulates kinase activity ( Fig. 1-18 ). Although it first appeared that HGFs activated JAK proteins in a rather promiscuous manner, some patterns have emerged ( Table 1-4 ). Thus receptors that are comprised of dimers such as the EPOR, G-CSFR, and TPOR associate with JAK2 (or to a lesser extent JAK1) in either a constitutive or ligand-dependent manner. Ligand binding and consequent clustering of receptor molecules leads to JAK2 aggregation and transphosphorylation at the lysine–glutamic acid–tyrosine–tyrosine (KEYY) site in the kinase activation loop. For example, the inactive EPOR exists as a homodimer each cytoplasmic domain of which is irreversibly bound to a molecule of JAK2. EPO binding to the extracellular domain of the receptor induces a conformational change that brings the JAK kinase domains together, resulting in rapid tyrosine transphosphorylation. In vitro experiments show that JAK2 phosphorylation leads to activation of its kinase function, which, taken together with evidence of association of JAK2 with the EPOR, suggests that JAK2 may act as the “master” protein tyrosine kinase that mediates the biologic response to EPO. Support comes from observations that a kinase-negative JAK2 acts as a dominant-negative suppressor of the proliferative response to EPO, and that the box1/box2 proximal cytoplasmic domain of the EPOR is the region that both binds JAK2 and is essential for receptor function. Furthermore, an acquired JAK2V617F mutation, found in almost all patients with polycythemia vera and about half of those with essential thrombocytosis and myelofibrosis, confers constitutive tyrosine kinase activity upon JAK2; it transforms hematopoietic cells when it is coexpressed with homodimeric cytokine type 1 receptors, and is sufficient for the development of polycythemia vera in murine recipients of transplanted JAK2V617F stem cells. A number of other substrates are phosphorylated in response to EPO, including nonreceptor kinases such as the p85 subunit of PI-3 K, substrates such as PLC-γ1 and the adaptor molecule Shc, and the tyrosine phosphatases hematopoietic cell phosphatase (HCP or PTP-1C) and Syp (also known as PTP-1D or SH-PTP2 ) that downregulate signal transduction; other kinases such as FES and Vav are also activated.
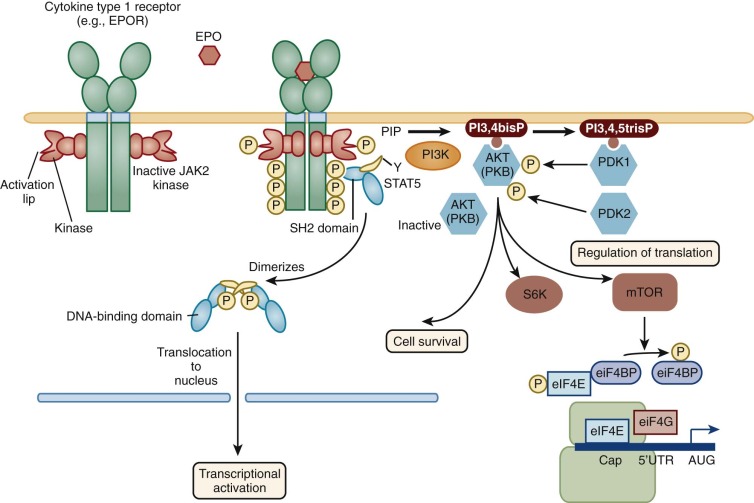
Receptor | JAK Family | JAK-Activated STATs | Other Nonreceptor Protein Tyrosine Kinases |
---|---|---|---|
KIT | JAK2 | PI3K | |
IFNRα/β | JAK1, Tyk2 | STAT1, STAT2, STAT3 | |
IFNRγ | JAK1, JAK2 | STAT1 | |
IL-3Rα (→βc) | JAK1 , JAK2 | STAT5, STAT6 | LYN, FYN, FES, TEC |
GM-CSFRα (→βc) | JAK1, JAK2 | STAT5 | FES |
IL-6Rα (→gp130) | JAK1 , JAK2 , Tyk2 | STAT1, STAT3 | HCK , BTK , TEC |
IL-2Rα (→β, γc) IL-4, IL-7, IL-9, IL-15Rαs (→γc) | JAK1 , JAK3 | STAT3, STAT5 STAT6 (IL-4 ) | LCK , FYN , LYN SYK |
EPOR | JAK2 | STAT5 | FES |
GCSFR | JAK1 , JAK2 | STAT3 | LYN , SYK , REL |
* Nonreceptor tyrosine kinases that associate with receptors are indicated in bold, and references are in superscript.
The other heterodimeric receptors appear to associate with and activate several JAKs (see Table 1-4 ). The beta common component of the IL-3/GM-CSF receptor is tyrosine phosphorylated after IL-3 or GM-CSF stimulation. The tyrosine kinase that is responsible for ligand-induced receptor phosphorylation could be JAK2 or JAK1, but the role of other recruited nonreceptor kinases such as LYN, FYN, and FES has not been clearly defined. Truncation mutants of βc show that the proximal cytoplasmic domain (amino acids 449 to 517) can induce JAK2 tyrosine phosphorylation and kinase activation, and this truncated receptor also retains the capacity to induce myc and pim-1 and to induce proliferation in serum-replete conditions but not serum-free conditions. Both gp130 and leukemia inhibitory factor receptor (LIFR) can associate with and activate JAK1, JAK2, and TYK2, but the pattern of such JAK family activation is distinct in different cell types.
Hematopoietic Growth Factor-–Induced Activation of STAT Proteins. The paradigm of the response to IFN has provided another major insight into a subsequent step in JAK family signal transduction. The kinase activities of TYK2 and JAK1 that are activated after IFN-α binding lead to phosphorylation of STAT1α/STAT1β, and STAT2. STAT proteins are characterized by an amino-terminal DNA-binding domain, a conserved SH2 domain, and a critical tyrosine at the carboxyl-terminus. Tyrosine phosphorylated STAT1α/β and STAT2 form heterodimers through their SH2 domains, bind to a 48-kD protein, translocate to the nucleus, and activate gene expression by binding to an interferon-stimulated response element (ISRE). STAT1α is also tyrosine phosphorylated (at Y ) after IFN-γ binds to the IFN-γR, and this event is associated with tyrosine phosphorylation of JAK1 and JAK2. STAT1α homodimerizes, translocates to the nucleus, and binds to the IFN-γ activated sequence (GAS), thereby transcriptionally activating genes that contain this sequence in their promoters.
The JAK proteins activated by HGFRs also lead to phosphorylation and DNA binding of STAT proteins; four additional members of the STAT family have been cloned: STAT3, STAT4, STAT5, and STAT6 (see Table 1-4 ). Thus ligand-induced activation of gp130, the signal-transducing component of the IL-6, LIF, IL-11, OSM, and CNTF family of receptors, leads to tyrosine phosphorylation of the JAK-Tyk family and activation of STAT3. STAT4 expression is restricted to spermatogonia as well as thymic and myeloid cells. STAT4 mRNA levels appear to decline when 32Dcl1 cells differentiate in either G-CSF or EPO. An ovine DNA-binding activity induced by prolactin called mammary gland factor was cloned and is named STAT5. STAT5 is activated by EPO and growth hormone as well as IL-3, GM-CSF, and IL-5. This 92-kD protein exists as two highly related proteins, STAT5A and STAT5B (96% identical), which are encoded by different genes. STAT6 is most closely related to STAT5 and is induced by IL-4.
Hematopoietic Growth Factor Signaling Through the RAS Pathway.
RAS is a “turnstile” through which signals from many receptors are routed. RAS guanosine triphosphate (GTP) associates with and activates RAF-1 and mitogen-activated protein kinase (MAPK). RAS is a 21-kD membrane-associated protein that cycles between the active GTP-bound forms and the inactive guanosine diphosphate (GDP)-bound forms in response to extracellular signals from various HGFRs, including KIT, IL-3, and GM-CSF receptors, T-cell receptor, IL-2R, and EPO R. Activation of RAS is mediated by guanine-nucleotide–releasing factors (GRFs) that catalyze the release of bound GDP and its exchange for GTP, while deactivation occurs by GTPase-activating proteins (GAPs) such as p120 GAP and neurofibromin that accelerate the intrinsic activity of RAS, leading to hydrolysis of GTP to GDP. The mammalian prototype for GRF-mediated RAS activation is the epidermal growth factor receptor (EGFR) the cytoplasmic domain of which bears intrinsic tyrosine kinase activity. After ligand-mediated dimerization, the EGFR is activated through autophosphorylation or transphosphorylation of tyrosine 1068. An adaptor protein, Grb2, binds to this phosphotyrosine through its SH2 domain. This in turn leads to the formation of a ternary complex of EGFR, Grb2, and a GRF called SOS, which is analogous to the Drosophila son of sevenless (SOS) protein. SOS contains a proline-rich region that recognizes two SH3 domains of Grb2, and the complex then activates membrane-bound RAS GDP to RAS GTP ( Fig. 1-17 ). The protooncogene vav also has RAS GDP/GTP nucleotide exchange activity and is activated through tyrosine phosphorylation after cross-linking of the T-cell antigen receptor–CD3 complex. With respect to the HGFRs, IL-2–mediated activation of the IL-2R leads to IL-2Rβ−chain tyrosine phosphorylation and association of the receptor with Shc, another adaptor protein that is characterized by both an SH2 domain and a second phosphotyrosine-binding (PTB) domain. Shc itself becomes phosphorylated on tyrosine, and this in turn leads to recruitment of Grb2 and SOS. The IL-3/GM-CSF–mediated signaling cascade involves phosphorylation of the beta subunit itself as well as Shc, with subsequent increased levels of RAS GTP and activation of RAF-1, MEK (also called MAPK kinase ), and MAPK, followed by induction of FOS and JUN (see Fig. 1-17 ). This activity was mapped to the cytoplasmic domain of βc between amino acids 626 and 763, whereas a more proximal domain (amino acids 449 to 517) retains the capacity to induce myc and pim-1. The PTB domain of Shc binds to tyrosine 577 of βc after JAK2-mediated phosphorylation of βc. RAS activation is important for both the proliferative and survival (prevention of apoptosis) functions. In the case of the EPOR, ligand-induced phosphorylation of Shc and the association of Shc with the EPOR has also been demonstrated; phosphorylated Shc associates with Grb2, and RAS and the MAP kinase pathway is activated. In summary, these three examples show that RAS is activated by a number of HGFRs, and that the RAS-RAF-MEK-MAP kinase pathway is important for proliferation and survival of hematopoietic cells.
The RAS pathway may also be important in certain leukemias. Neurofibromin, encoded by the NF-1 gene, is mutated in patients with autosomal dominant neurofibromatosis type 1 (NF-1). NF-1 shows sequence homology with yeast and mammalian GAP genes. Children with neurofibromatosis have an increased risk of juvenile myelomonocytic leukemia (JMML), monosomy 7 syndrome, and acute myeloblastic leukemia. Importantly, leukemic cells from children with NF-1 and myelodysplastic syndrome show loss of the normal NF-1 allele, thus implicating NF-1 as a tumor suppressor gene. NF-1 GAP activity is significantly lower in cell lysates prepared from NF-1–associated leukemias than in normal bone marrow or non–NF-1 leukemic lysates. Bone marrow mononuclear cells from patients with JMML show an increase in CFU-GM in response to GM-CSF but not in response to IL-3. Interestingly, fetal liver cells from mice that are rendered null for the NF-1 gene show similar hypersensitivity to GM-CSF, indicating that NF-1 GAP may play a crucial and specific role in the response of myeloid cells to GM-CSF. NF-1 −/− mice die in utero around day 13.5 to 14.5 from complex cardiac defects, but transplantation of fetal liver cells on day 11.5 to 13.5 into lethally irradiated recipients produces a myeloproliferative syndrome similar to that of the human disease. BCR-ABL is a chimeric oncogenic protein that shows dysregulated tyrosine kinase activity and is implicated in the pathogenesis of Philadelphia chromosome–positive chronic myeloid leukemia. A phosphorylated tyrosine (Y177) in the BCR first exon binds to the SH2 domain of Grb2 and activates RAS. Mutation of the Y177 BCR-ABL to phenylalanine abolishes Grb2 binding and abrogates both BCR-ABL–induced RAS activation and transformation of primary bone marrow cultures. In summary, RAS dysregulation may contribute to the increased proliferation that characterizes these two chronic leukemias.
Phosphatases and Receptor Signaling. The C-terminal domain of the EPOR has a negative regulatory role. It is likely that this effect is mediated by a phosphatase that dephosphorylates kinase-associated tyrosine(s) in this region, as has been shown for the IL-3R. There is a fascinating report of a Finnish family with dominantly inherited benign erythrocytosis. The proband, an Olympic cross-country skier, has a mutation in the EPOR that introduces a premature stop codon and generates a receptor lacking the C-terminal 70 amino acids. This mutation cosegregates with the disease phenotype in this large family. Recent data show that the nontransmembrane protein tyrosine phosphatase SH-PTP1 (also called SHP-1, HCP, and PTP1C) associates via its SH2 domain with tyrosine-phosphorylated EPOR. Mutational analysis mapped the binding site most probably to Y429, and this tyrosine is deleted with the C-terminal truncation of the EPOR in the Finnish family with benign erythrocytosis. Factor-dependent cells that express a Y429,431F mutant EPOR show increased sensitivity to EPO, as do cultured erythroid progenitors from patients with benign erythrocytosis. CFU-E from mice that lack or have impaired SH-PTP1 activity (motheaten or motheaten viable) show a similar increased sensitivity to EPO. Therefore, EPO-induced activation and subsequent tyrosine phosphorylation of the EPOR leads to recruitment of SH-PTP1, which then plays a major role in terminating the EPO signal, possibly through dephosphorylation of JAK2 or other tyrosine kinases.
Another nonreceptor protein tyrosine phosphatase called SH-PTP2 or SHP2, encoded by the PTPN11 gene, has 50% to 60% identity with SH-PTP1 in both SH2 and catalytic domains. Despite this similarity, SH-PTP2 appears to be a positive regulator of some growth factor pathways, in particular RAS. Approximately 50% of patients with Noonan syndrome (NS), a developmental disorder characterized by short stature, dysmorphic features, and cardiac defects, have germline mutations in SH-PTP2, and 35% of patients with JMML but not NS have somatic mutations in this gene. Some patients with NS develop JMML, and it appears that the mutations in NS/JMML differ from those found in de novo nonsyndromic JMML, and differ in the degree to which they activate the phosphatase.
Other Inhibitors of Hematopoietic Growth Factor Signaling. Another family of negative regulators is made up of the suppressors of cytokine-signaling (SOCS) proteins. SOCS-1 was discovered by three groups, contains an SH2 domain, and has sequence and structural similarities to an immediate early gene called CIS (cytokine-inducible SH2–containing protein). It is one of an eight-member gene family (SOCS-1 to SOCS-7 and CIS) that is characterized by a central SH2 domain and a C-terminal conserved motif called a SOCS box . Unlike the constitutively expressed phosphatases, SOCS expression is induced by many cytokines, including IL-3, EPO, TPO, IL-6, and LIF, and the proteins are expressed in hematopoietic and other tissues. SOCS-1, expressed predominantly in the thymus, interacts directly with the phosphorylated activation loop of (activated) JAKs, and its N-terminus is thought to inhibit adenosine triphophate (ATP) binding to the activation cleft. Subsequently, the SOCS box is hypothesized to target the SOCS/signaling complex to the proteosome for degradation by recruiting components of the proteasome machinery, including elongins B and C and Cullin 2. SOCS-3 has been shown to inhibit signaling by a different mechanism, which is binding directly to activated receptors and to JAKs without inhibiting JAK activity, whereas CIS can bind to phosphorylated tyrosines on the EPO receptor and thereby inhibit STAT5 signaling.
SOCS knockouts have provided insight into the important physiologic roles of some of these proteins. SOCS-1 −/− mice die before weaning with fatty degeneration of the liver, reduced thymic size, lack of T and B cells, and monocytic infiltration of organs. This phenotype is similar to mice with elevated IFN-γ, and indeed SOCS-1 −/− mice have increased IFN-γ levels; rescue of the animals can be achieved with antibodies to IFN-γ or by crossing mice with IFN-γ −/− mice. SOCS-2 −/− mice are normal at birth but then become significantly larger than wild-type mice, with thickening of the dermis due to excess collagen deposition. Therefore SOCS-2 appears to play an important role in postnatal growth, possibly through the growth hormone/insulin-like growth factor-1 axis. SOCS-3 −/− mice are hematopoietically most interesting in that they die at embryonic day 12 to 14 because of massive erythrocytosis. Progenitor cells appear to be hyperresponsive to IL-3 and EPO. Thus SOCS-3 plays a crucial role in inhibiting fetal erythropoiesis. Although mice in which CIS has been deleted show no abnormalities, overexpression of CIS induces a phenotype similar to that of Stat5a −/− or Stat5b −/− animals.
Biology of Hematopoiesis
Stem Cells
The formed elements of the blood in vertebrates, including humans, continuously undergo replacement to maintain a constant number of red cells, white cells, and platelets. The number of cells of each type is maintained in a very narrow range in the physiologically normal adult, approximately 5,000 granulocytes, 5 × 10 6 red blood cells, and 150,000 to 300,000 platelets/µL of whole blood (see Appendix for normal values in infancy and childhood). In this section we examine the normal regulatory mechanisms that maintain a balanced production of new blood cells. These regulatory systems are far from completely understood, but present evidence strongly supports the following basic principles, schematically depicted in Figure 1-7 :
- 1.
A single pluripotent stem cell is capable of giving rise to many committed progenitor cells. These committed progenitors are destined to form differentiated recognizable precursors of the specific types of blood cells.
- 2.
The pluripotent stem cell is capable of self-renewal. The committed progenitor cells are limited in proliferative potential and are not capable of indefinite self-renewal. In addition to their limited proliferative potential, committed progenitors also “die by differentiation” and their numbers depend on influx from the pluripotent stem cell pool.
- 3.
The proliferative potential and differentiation of stem cells and committed progenitors may be influenced by niche cells or factors derived from them. Thymus-derived lymphocytes appear to contribute to the induction of spleen colony formation in irradiated recipients. The hematopoietic role of thymus-derived cells is further supported by experiments demonstrating the defective restorative capacity of bone marrow from congenitally athymic or neonatally thymectomized mice, or rigorously T-depleted human bone marrow.
- 4.
Committed progenitor cells are capable of response to humoral- or marrow stroma–derived regulators, some produced in reaction to the circulating levels of a particular differentiated cell type. In this response, they proliferate and differentiate to form the recognizable blood cells. Under this type of control, amplification of production occurs at the committed progenitor cell level. Most of the regulatory molecules are produced by hematopoietic accessory cells in close proximity to progenitor cells. As differentiation proceeds, sensitivity to the late-acting factor increases. This late-acting regulator may serve to protect the highly proliferative but numerically limited immature progenitor compartment from exhaustion or death by differentiation under these stress conditions.
- 5.
Hematopoietic differentiation requires an appropriate microenvironment. In normal humans, this environment is confined to the bone marrow, whereas in the mouse the microenvironment includes both the spleen and the bone marrow. The existence of the Steel (Sl) strains of mutant mice that exhibit a deficiency in the hematopoietic microenvironment (see below) suggests that the interactions between hematopoietic progenitors and the bone marrow microenvironment involve very specific molecular mechanisms. A number of early hematopoietic cells, including the pluripotent stem cells and certain committed progenitor cells, have been demonstrated in the circulation of normal individuals and/or experimental animals. The capacity of HSCs to negotiate nonhematopoietic tissues via the circulation is especially significant in relation to bone marrow transplantation, which is carried out by infusion of bone marrow or blood cells from the donor into the circulation of the recipient. For example, blockade of hepatic asialoglycoprotein receptors enhances stem cell engraftment in murine spleen and marrow. There is also convincing evidence that murine stem cells (CFU-S) and progenitors (CFU-GM) express “homing” or adhesive lectin receptors that bind to the mannose and/or galactose presented on stromal cells. For example, homing of transplanted mouse progenitors can be blocked by synthetic neoglycoproteins with these specificities, suggesting that stromal cells are likely to express these sugars. SDF-1/chemokine (CXCR4) signaling, adhesion molecules, including VLA-4/VCAM-1 and CD44/hyaluronic acid interactions, are also important in the regulation of homing.
If only 10% to 20% of stem cells are in cycle in the resting state, what is their role with regard to self-renewal versus differentiation, and what is the role of the noncycling stem cells? The hypothesis that a series of stem cells may contribute clones successively to maintain hematopoiesis throughout the life span of an individual was first advanced by Kay. Support for this hypothesis comes from transplant studies of recipients of small numbers of bone marrow cells and from experiments in which mixtures of fetal liver cells from different inbred mouse strains were introduced into W-mutant fetuses. W mutants exhibit a genetic deficiency of HSCs (as well as germ and follicle cells) (see below). Long-term monitoring showed clonal dominance followed by a decline of cells of particular genotypes.
The use of retroviral-mediated gene transfer to mark HSCs at first lent support to this hypothesis. Analysis of the viral integration patterns at intervals after transplantation documented concurrent contributions of small numbers of stem cells to hematopoiesis, with changing patterns over time. These conclusions are not supported by the work of Harrison, who measured variances at successive intervals in recipients of marrow mixtures from two congenic donors. The high correlations observed in this study suggest that the transplanted stem cells continuously produce descendants. However, if 10 to 20 (or more) clones are contributing to hematopoiesis simultaneously, then even with clonal succession one might expect variances between two alloenzymes to be minimized at sequential samplings. Furthermore, a longer-term analysis of viral integration patterns in multiple-lineage progeny of stem cells transplanted into mice shows that integration patterns are unstable initially but then stabilize and maintain consistency over many months. Although this consistency does not appear to support the clonal succession hypothesis, the contributory role of unmarked stem cells in these experiments cannot be assessed. Furthermore, it is not possible to determine whether the immediate progeny of the transplanted (and retrovirally marked) stem cells might not all still have self-renewal capacity and subsequently contribute to hematopoiesis successively. Such clones would carry the same integration marker. A problem with the transplant approach discussed above is that the preparation required for successful transplantation may perturb hematopoiesis and complicate interpretation of the results. A resolution of the problem came from studies in which a nontoxic dose of bromodeoxyuridine in drinking water was fed to mice. Bromodeoxyuridine is incorporated into DNA by proliferating cells, and measurement of incorporation rates into primitive stem cell populations over time can be used as a monitor of proliferative history. Under steady-state conditions, approximately 5% of the LTR-HSCs were in cycle and another 20% in the G 1 phase, with approximately 8% entering the cell cycle per day. By 6 days, 1 month, and 6 months, 50%, 90%, and 99%, respectively, of the stem cell pool had incorporated the label. Because the size of the stem cell pool remains constant, these data indicate that 50% of dividing LTR-HSCs must self-renew; this could be accomplished by asymmetric division or by symmetric division in which half the cells give rise to LTR-HSCs and the other half to differentiating cells. More recently, fluorescent marking studies and bar-coding strategies have suggested that significant numbers of clones participate in hematopoiesis.
Transcription Factors and Stem Cells
Although the factors that control the “decisions” of stem cells to undergo either self-renewal or commitment to differentiate down one of the alternate lineage pathways are poorly understood, in recent years nuclear transcription factors have been characterized that play a role in stem or early progenitor cell proliferation and lineage commitment. The Tal1, LMO2, and GATA family of transcription factors is important in this regard. In particular, tal-1/SCL, a basic helix-loop-helix (bHLH) transcription factor, is expressed in biphenotypic (lymphoid/myeloid) and T-cell leukemias and in both early hematopoietic progenitors and more mature erythroid, mast, megakaryocyte, and endothelial cells. Targeted disruption of the Tal-1/Scl gene leads to death in utero from absence of blood formation, and lack of in vitro myeloid colony formation suggests a role for this factor very early during hematopoiesis at the pluripotent or myeloid-erythroid stem cell level. Another transcription factor implicated in T-cell ALL is the LIM domain–only nuclear protein (Lmo2). Mice that lack this factor die in utero and have the same bloodless phenotype as the Tal1 −/− animals. Interestingly, although Lmo2 does not show sequence-specific DNA binding, immunoprecipitates in erythroid cells show that Lmo2 exists in a complex with Tal1, suggesting a physiologic interaction in vivo. GATA2 is expressed in the regions of the Xenopus and zebrafish embryos that are fated to become hematopoietic, and is highly expressed in progenitor cells. Overexpression of GATA2 in chicken erythroid progenitors leads to proliferation at the expense of differentiation. Targeted disruption of the Gata2 gene by homologous recombination in embryonic stem cells leads to reduced primitive hematopoiesis in the yolk sac and embryonic death by day 10 to 11. Definitive hematopoiesis in liver and bone marrow is profoundly reduced, with loss of virtually all lineages, and in vitro differentiation data show a marked deficiency of SF-responsive definitive erythroid and mast cell colonies and reduced macrophage colonies, suggesting that GATA2 serves as a regulator of genes that control HGF responsiveness or proliferation of stem and/or early progenitor cells. Dominant mutations in GATA2 have recently been described in the monoMAC syndrome, characterized by nontuberculous mycobacterial infections (frequently Mycobacterium avium complex [MAC] infections), fungal infections, disseminated human papillomavirus infection, and pulmonary alveolar proteinosis. Patients have a marked reduction of monocytes, NK cells, and B cells, and can develop myelodysplasia and leukemia.
These data contrast with the later time of embryonic death (day 15) from anemia during the midfetal liver stage in mice with targeted disruption of Myb and Rb and severe forms of W and Sl mutations (see below). Myb has been shown to be absolutely required for normal hematopoietic production during embryogenesis. Embryonic erythropoiesis in Myb knockout mice is normal, but there is complete failure of erythropoiesis in fetal liver. Progenitors of other lineages, but not megakaryocytes, were also decreased, indicating that Myb is required for early definitive cellular expansion. Furthermore, a knockdown allele of Myb shows that suboptimal levels of Myb favor macrophage and megakaryocyte differentiation whereas higher levels are particularly important for erythropoiesis and lymphopoiesis. An ethylnitrosourea (ENU)-induced allele of Myb (M303V) affects the transactivation domain of the protein that interacts with the transcriptional coactivator p300; recessive mice ( Myb M303V/M303V ) demonstrate increased platelet production, anemia, absence of eosinophils, and defects in B-cell and T-cell lymphopoiesis. These animals were found to have an increase in transplantable HSCs, indicating that Myb has a negative role in HSC self-renewal. Thus Myb appears to have a negative role in HSC and megakaryocyte progenitor proliferation and positive effects on erythropoiesis, eosinophil production, and lymphopoiesis.
Loss of function of the runx1 (or AML1 ) gene, which encodes the alpha subunit of the heterodimeric core-binding factor (CBF), results in fetal death by day 12.5 because of failure of production of all definitive hematopoietic lineages. CBF recognition sequences are present in the IL-3, GM-CSF, M-CSFR, and T-cell antigen receptor promoters. The runx1 gene is frequently rearranged in acute myelogenous leukemia (AML) and childhood acute lymphoblastic leukemia (ALL), and is expressed in myeloid and lymphoid cells. Runx1 has been shown to interact with a number of repressors, including GROUCHO domain factors. Runx1 is also a regulator of HSC production in the aorta-gonads-mesonephros region, as well as in adult stem cell homeostasis. A rescue of runx1 by a Tek (Tie2) promoter rescues hematopoiesis during development.
There are GATA1 PU.1 interactions regulating an erythroid/myeloid decision, and there are many other transcription factor interactions, such as CEBPa induction of PU.1 in the myeloid system. Such competition for transcription factors and the regulation of stem cell differentiation appears to be a recurring mechanism in the hematopoietic system. Manipulation of the process actually leads to altered hematopoiesis that can favor a single lineage. This involves a cell fate change rather than a proliferative event.
Homeobox ( HOX ) genes encode transcription factors involved in the establishment of body pattern and tissue identity during development. The human genes occur in four clusters, HOX A to D, on chromosomes 7, 17, 12, and 2, respectively. HOXA and HOXB genes are expressed in CD34+ cells and downregulated as these cells mature. Intriguingly, murine bone marrow cells engineered to overexpress Hoxb4 by retrovirus-mediated gene transfer show a dramatic upregulation in stem cell activity both in vitro and in vivo, although it may not be required for normal hematopoiesis. Serial transplantation studies showed a greatly enhanced ability of Hoxb4 -transduced bone marrow cells to regenerate the most primitive HSC compartment, resulting in fiftyfold-higher numbers of transplantable totipotent HSCs in primary and secondary recipients, compared with serially passaged control cells. This in vivo expansion of Hoxb4 -transduced HSCs was not accompanied by identifiable anomalies in the blood of these mice, suggesting that Hoxb4 is an important regulator of very early but not late hematopoietic cell proliferation. Recent data show that ectopic expression of Hoxb4 in yolk sac and embryonic stem cells confers long-term engraftment capacity in primary and secondary recipients. Daley and colleagues reported that nuclei from Rag2 knockout (Rag2 −/− ) tail cells could be transferred into enucleated oocytes, and embryonic stem (ntES) cell lines could be derived from the resulting blastocysts. The Rag2 mutation was then corrected by homologous recombination. Insertion of Hoxb4 into the corrected embryonic stem cells allowed their expansion into transplantable HSCs. These cells were able to partially correct the defect in immunoglobulin production in Rag2 −/− recipients after bone marrow transplantation. The HOX pathway has been expanded to include HOX A7 and A9 as major regulators of hematopoiesis and leukemia formation. The posterior Hox genes therefore drive HSC production; this was highlighted by the finding that mutants of Cdx4, a controller of HOX gene expression, lack HSCs in the zebrafish and Cdx controls Hox gene expression for the formation of HSCs.
The Gfi1 locus was originally identified as an oncogene causing lymphoma based on retroviral insertion. Gfi1 contains an internal SNAG domain and a six–zinc-finger DNA-binding domain. There are two nearly identical genes called Gfi1 and Gfi1b . Gfi1 is restricted to the lymphoid system and neutrophils, whereas Gfi1b seems to be distributed throughout the entire hematopoietic system. Gfi1 knockout animals have a severe reduction in lymphoid progenitors. This leads to an early lack in both CD4+ and CD8+ cells. In addition, IL-7 receptor signaling is suppressed. Gfi1 is a major regulator of neutrophil maturation. Normal neutrophils are absent in the knockout animals but eosinophils are present in normal numbers. The response of neutrophils to G-CSF is preserved, but differentiation does not occur. There is a significant interaction of Gfi1 with other transcription factors, including PU.1, Cebpβ, and Cebpε, which regulate neutrophil maturation.
Gfi1 also has been shown to be involved in HSC self-renewal. Gfi1 knockout animals actually increase and outcompete other cells. This leads to stem cell exhaustion. Gfi1b may also regulate stem cell homeostasis. The HSCs appear to be more active in terms of their cell cycle and have increased proliferation rates. It is clear that p21 is likely one of the mediators of Gfi1 in HSC production. There is also modulation of the STAT3 activity as a consequence of the interaction of Gfi1 with PIAS-3, a factor that simulates transcription factors. This is interesting because other genes such as Tal1 , Runx1 , Notch 1 , Notch 2 , RBPJ , integrin-1, Hoxb4 , and β-catenin ( CTNNB ) are dispensable for most HSC activities, whereas p21 ( CDKN1A ), Bmi 1, tel1, Cebp, PU.1, and Gfi are actually required.
Growth Factors and Stem Cells
HGFs also appear to influence at least some classes of stem cells. Analysis of the actions of HGFs on “purified” murine or human stem and progenitor cells has led to several major conclusions. These conclusions should be interpreted in light of the fact that, to date, even the most highly purified “stem cell” fractions are still heterogeneous with respect to content of LTR-HSC, HSC with short-term reconstituting potential, and lineage-committed progenitors. HGFs such as SCF and perhaps IL-3, GM-CSF, and G-CSF can independently support the survival of murine and/or human stem cell populations. Of all of these HGFs, however, only SCF has been shown to enhance the survival of murine LTR-HSCs, and no single HGF or combination of HGFs has unequivocally shown the capacity to induce significant self-renewal of LTR-HSCs. Mice deficient in IL-6 show a reduction in CFU-S and IL-6 −/− bone marrow cells fail to contribute to long-term hematopoiesis after serial transfer. In addition to its effect on megakaryocyte and platelet formation, TPO is also vital for stem cell production. Mice that lack the receptor for TPO, Mpl, show reduced numbers of blast colonies and CFU-S, and fail to compete effectively with normal stem cells for reconstitution of hematopoiesis in irradiated recipients, even when transplanted in tenfold excess. Furthermore, both murine and human repopulating activity segregates with primitive cells that express MPL. In the human system, information on HSC self-renewal is not available, because no human assay measures this property.
Analysis of mutations that affect the function of stem cells and the hematopoietic microenvironment through HGF interactions has provided important insights. Two murine mutations have been characterized in some detail, the Steel (Sl) and dominant white spotting (W) mutations. Genetically affected mice that are defective at these loci have a severe macrocytic anemia associated with increased radiation sensitivity, lack of pigmentation, poor gastrointestinal motility, and sterility. Although phenotypically similar, these mutations map to different chromosomes (W to chromosome 5, Sl to chromosome 10) and have distinct hematologic characteristics. The W mutation, which is best characterized at the molecular level, results in a functional deficiency of HSCs and, particularly, erythroid progenitors. Transplantation of normal bone marrow into homozygous W recipients completely corrects the hematopoietic abnormalities, whereas transplantation of W bone marrow into normal mice fails to reconstitute hematopoiesis. The W gene is allelic with the Kit protooncogene, which, as discussed above, is known to be a member of a tyrosine kinase receptor family. Several variants of the W mutation have been shown to be phenotypic expressions of specific mutations of the Kit gene, and the severity of the phenotypic changes associated with specific alleles correlates with the degree of functional impairment of kinase activity.
In contrast, the Steel mutation affects the function of the cells of the microenvironment. Sl/Sl d bone marrow cells are capable of reconstituting hematopoiesis in irradiated normal mice, whereas transplantation of normal bone marrow into Sl/Sl d animals fails to cure the defect. A deeper insight into the molecular nature of this defect was provided by the purification of a factor that is active on mast cells and on mouse bone marrow that has been exposed to 5-FU (enriched for primitive stem cells but depleted of mature progenitors). Labeling studies with the purified recombinant protein demonstrate that it is the ligand for Kit. Furthermore, administration of the factor to Sl/Sl d mice corrects their macrocytic anemia and repairs their mast cell deficiency. Less severe mutations at the Sl locus, such as Sl d , are associated with smaller deletions at the Sl locus. The gene encodes a protein that exists in both transmembrane and secreted forms. Recent studies have shown that SCF is expressed by HSCs and perivascular stromal cells in the marrow niche, but expression by endothelial cells and leptin receptor perivascular cells is critical for HSC survival.
Recently, other growth factor pathways that are important during embryogenesis and have been subverted in certain cancers have also been implicated in stem cell self-renewal. Notch receptors (four members, Notch 1 to 4) are expressed on the surface of a variety of hematopoietic cells as proteolytically cleaved dimers comprising an EGF-repeat–rich extracellular domain and a transmembrane/intracellular domain that contains two motifs (RAM and ANK) that can bind to a transcriptional repressor CBF1/RBPJκ (see Kojika and Griffin for a detailed review). After ligand binding (three ligands, Delta, Jagged1, and Jagged2), the transmembrane/intracellular domain is cleaved to release the active intracellular domain of Notch (ICN), which translocates to the nucleus, binds to CBF1/RPBJκ, and transactivates genes such as Hairy and Enhancer of Split (HES)-1, a bHLH protein that suppresses transcription of N-box (CACNAG) target genes. Human Notch 1 was first identified as a gene called TAN-1 that is involved in some T-cell leukemias, in which it generates an intracellular activated form of Notch 1 (ICN-1). Remarkably, overexpression of ICN-1 in murine stem cells leads to immortalization of cytokine-dependent cell lines that can generate lymphoid or myeloid progeny in vitro and in vivo, suggesting a role for Notch 1 in stem cell self-renewal. Activating mutations in Notch have been found in many T-cell leukemias. Addition of soluble Jagged1 to cultures of human purified stem and progenitor cells also increased NOD/SCID repopulating activity. A clinical trial was recently completed using soluble Delta as a means of expanding hematopoiesis in vitro. Cord blood was treated with the soluble Delta, and the expanded progenitors were then infused into the patient. There was no effect on stem cells. This treatment enhanced neutrophil recovery following preparative chemotherapy and irradiation.
A second signaling pathway that may be involved in stem cell self-renewal involves the Sonic hedgehog protein. Shh activates the Smoothened receptor (Smo) by binding to Patched, its inhibitor. Smo initiates signaling that regulates transcription factors of the Gli family, which modify expression of genes that encode BMP-4 and its inhibitor Noggin. Shh has recently been shown to induce expansion of human NOD/SCID repopulating cells, possibly through regulation of BMP-4. Last, Wnt signaling may also be involved in stem cell self-renewal. Wnt signals through the receptors Frizzled and lipoprotein receptor–related protein (LRP) to activate a cytoplasmic protein called Dishevilled, which in turn stabilizes β-catenin, a transcriptional coactivator that associates with the Tcf/LEF family of transcription factors. Without Wnt signaling, β-catenin is normally destabilized by a cytoplasmic complex that comprises Axin, adenomatous polyposis coli, and glycogen synthase kinase 3β (GSK-3β). Overexpression of activated β-catenin in highly purified murine HSCs appears able to expand the pool of transplantable cells. Controversy still exists about the role of wnt signaling during homeostasis, but it seems to have a role during stress. Mammalian target of rapamycin (mTOR) may cooperate with wnt at homeostasis. The wnt pathway established different responses based on level of activation.
Two models have been proposed to explain the mechanisms that influence the choice of stem cells between self-renewal and commitment. A stochastic model was proposed by Till, McCullough, and Siminovitch. In this model, self-renewal or differentiation is considered to occur in a random or stochastic manner, dictated only by a certain probability. Because no extrinsic source of stem cells exists, under steady-state conditions the overall probability of stem cell division resulting in self-renewal must be 0.5; probabilities less or greater than this value would lead to progressive stem cell depletion (aplasia) or expansion (leukemia), respectively. The commitment of stem cells could occur symmetrically, where overall, half the stem cells divide to produce progeny both of which enter a differentiation pathway, and the other half of the stem cells generate progeny that are both stem cells. Alternatively, asymmetric division would give rise to one stem cell and one cell committed to differentiate. Analysis of single cells from blast cell colonies has shown that hematopoietic cells can divide asymmetrically; these micromanipulated single cells give rise to colonies that comprise almost all possible lineage combinations. Single-cell analysis of purified human CD34+CD45RA lo CD71 lo provides evidence for quiescent survival, self-renewal, and asymmetric division. These data provide support for a stochastic mechanism to explain both commitment and restriction of differentiation potential. The best purification strategies still allow only one of two cells to be an HSC, and paired daughter analysis has some caveats with regard to lineage tracing. During the regeneration of hematopoietic tissues after injury by irradiation or cytotoxic drugs, the probability of generating stem cells must increase. How this probability is altered is one of the outstanding unanswered questions in hematopoiesis.
The osteoblastic niche in bone marrow plays a role in stem cell self-renewal, whereas the sinusoidal endothelial niche regulates proliferation, differentiation, and mobilization. These functional differences suggest a mechanism for the external control of stem cell expression. This observation and others related to the morphology of the focal development of hematopoietic islands attached to adherent cells have led to postulation that HSCs reside within stromal cell “niches” that play a vital role in supporting stem cell survival, perhaps by intimate cell contact (see Fig. 1-5 ). Interestingly, a recent study showed that deletion of Dicer1, an RNAseIII endonuclease important for microRNA biogenesis, in osteoprogenitors led to myelodysplasia (MDS) and acute myeloid leukemia (AML). Most intriguing, the dysplastic or leukemic hematopoietic cells retained Dicer1 expression. Subsequent gene expression studies showed reduced expression of the SBDS gene, which is mutated in 90% of patients with Shwachman-Diamond syndrome, who also have an increased risk of development of MDS and AML. Thus this study suggests, surprisingly, that stromal cell dysfunction may have an important role in secondary oncogenesis.
Whether a stochastic mechanism or one influenced by environmental cues dictates the outcome of the stem cell decision to commit to one or the other hematopoietic lineage is also uncertain. Recent experiments suggest that cross-antagonism among lineage-specific transcription factors may provide a mechanistic explanation for lineage specification. Forced expression of the erythroid-specific factor GATA1 in myelomonocytic cells perturbs their ability to differentiate, and evidence is accumulating that this is due to direct physical interaction and cross-antagonism of PU.1, the myeloid transcription factor, and GATA1; GATA1 inhibits PU.1 by preventing it from interacting with its cofactor JUN, whereas PU.1 prevents GATA1 from binding to DNA. In Down syndrome patients, there are mutations in GATA1, which lead to a truncated protein missing its N-terminus. This appears to give competitive advantage to a yolk sac/fetal liver progenitor in the genesis of leukemia and transient myeloproliferative disorder. Another example is the antagonism between FOG1 and C/EBP-β in an eosinophilic cell line, in that enforced expression of FOG1 blocks C/EBP-β mediated eosinophil differentiation. It is possible that lineage-specific transcription factors not only have potent inductive effects but also exert blocking effects on alternate lineage choices. The observation that forced expression of C/EBP-β in pancreatic cells promoted their differentiation into a hepatic phenotype raises the intriguing possibility that this mechanism underlies stem cell plasticity as well (reviewed by Cantor and Orkin ).
Erythropoiesis
It is well established that the level of oxyhemoglobin and the rate of delivery of oxygen to the tissues is the fundamental stimulus of erythropoiesis. In species that package hemoglobin in erythrocytes, erythropoietin mediates the response to oxygen demand and does so by interacting with specific receptors that are found on the surface of committed erythroid progenitor cells and erythroblasts. Insight into the mechanism by which hypoxia induces a transcriptional increase in the expression of the Epo gene has come from the identification of the hypoxia-inducible transcription factor HIF-1α.
It is believed that BFU-E do not directly give rise to erythroblasts in vivo except under conditions of extreme anemic stress. Instead, they mature to single erythroid colony-forming units, or CFU-E, which divide in vivo. Under the influence of lower concentrations of erythropoietin these CFU-E form single, relatively small colonies of proerythroblasts at about 1 week in vitro. Many (at least half) of CFU-E will form erythroid colonies in vitro in response to erythropoietin alone. They do not require the additional presence of either GM-CSF or IL-3, having differentiated beyond this requirement. All proerythroblasts can differentiate in the presence of erythropoietin alone. In the steady state, the number of human CFU-E exceeds the number of BFU-E by a factor of 10. CFU-E are larger than BFU-E, and because a higher fraction of CFU-E are in the S phase of DNA synthesis than are BFU-E, the former exhibit a higher rate of “suicide” in response to exposure to tritiated thymidine. The membranes of mature BFU-E and CFU-E are CD34+ and HLA-DR+, and various negative and positive selection methods may be used to purify them from sources such as bone marrow and human fetal liver. They have the appearance of lymphoblasts.
The bone marrow of an adult mouse contains about 500 CFU-E per 10 5 nucleated cells. In response to anemic stress, as in hemorrhage or hemolysis, nearly the entire burden of accelerated reticulocyte production is borne by the rapid erythropoietin-dependent influx into the proerythroblast pool from the progenitor compartment. This produces an expanded proerythroblast pool. Little or no increase in the mitotic rate of recognizable erythroid precursors occurs. Instead, the late BFU-E and CFU-E proliferate in response to the engagement of their erythropoietin receptors by the hormone and, as well, differentiate to proerythroblasts and beyond. In normal murine marrow, following experimental hemorrhage or hemolysis, the CFU-E frequency of about 500 per 10 5 nucleated cells increases to 1000 to 2000 CFU-E per 10 5 nucleated cells. In contrast, hypertransfused mice exhibit a reduced number of CFU-E per 10 5 nucleated marrow cells (values between 10% and 20% of normal have been reported).
During stress erythropoiesis induced by anemia, the orderly progression from immature BFU-E through CFU-E to proerythroblast is interrupted as high erythropoietin levels appear to permit or induce differentiation of immature progenitors to proerythroblasts. In the rhesus monkey this premature terminal differentiation may account for the marked increase in fetal hemoglobin content and F cells seen in simian stress erythropoiesis. The ability of human progenitors to generate erythroblasts capable of synthesizing large quantities of fetal hemoglobin is less pronounced. Thus the accumulation of F cells in peripheral blood in response to anemia is relatively small. Such red cells are usually macrocytic and carry the i antigen as well, but these two characteristics may relate to a short transit time through the marrow in response to erythropoietin rather than to an intrinsic characteristic of fetal hemoglobin-containing cells themselves (see below). F cells are also present in very small numbers in the blood of normal individuals, but these cells are not macrocytic and do not bear i antigen. F cell progenitors can be detected easily in the bone marrow of patients with various hemoglobinopathies and even in normal marrow.
Transcription Factors and Erythropoiesis
As discussed above, targeted disruption of the Tal1 , Lmo2 , and Gata2 genes leads to embryonic lethality because of failure of blood production, probably at the level of the HSC. GATA1 expression is limited to multipotent progenitors and erythroid, megakaryocyte, mast, and eosinophil lineages. Analysis of chimeric mice injected at the blastocyst stage with Gata1 −/− embryonic stem cells shows a failure of a contribution of the Gata1 −/− cells to erythrocytes but development into other hematopoietic lineages, as well as other tissues. Differentiation assays in vitro show proerythroblast arrest and apoptosis of these cells. A transcriptional cofactor of GATA1, Friend of GATA1 (FOG1), was discovered and found to be essential for erythropoiesis and megakaryopoiesis. Evidence that this interaction is essential in vivo comes from studies of a family with severe dyserythropoietic anemia and thrombocytopenia. Affected family members have a mutation in GATA1 that disrupts its interaction with FOG1 without affecting DNA-binding affinity. The EKLF transcription factor was found as an activity bound to the CACCC site of the β-globin promoter. It controls globin expression and some aspects of erythroid development. The NF-E2 protein complex binds to the locus control region (LCR) to regulate globin gene transcription; it is a dimer of a tissue-restricted (erythroid/megakaryocyte/mast cell) cnc domain subunit of 45 kD and a smaller, widely expressed subunit of 18 kD, and is essential for platelet, but not erythrocyte, production.
Growth Factors and Erythropoiesis
The regulation of the proliferation and maturation of erythroid progenitors depends on interaction with a number of growth factors. The availability of pure recombinant growth factors, the enrichment of target progenitor cells, and the use of defined “serum-free” culture conditions have provided insights into the role of these factors during hematopoiesis.
Erythropoietin (EPO) is essential for the terminal maturation of erythroid cells. Its major effect appears to be at the level of the CFU-E during adult erythropoiesis, and recombinant preparations are as effective as the natural hormone. These progenitors do not require “burst-promoting activity” in the form of IL-3 or GM-CSF, and their dependence on EPO is emphasized by the observation that they will not survive in vitro in the absence of EPO. Because most CFU-E are in cycle, their survival in the presence of EPO is probably tightly linked to their proliferation and differentiation to mature erythrocytes. EPO also acts on a subset of presumptive mature BFU-E, which also require EPO for survival and terminal maturation. A second subset of BFU-E, presumably less mature, survive EPO deprivation if IL-3 or GM-CSF (IL-3 more than GM-CSF) is present. When EPO is added to these cultures on day 3 these BFU-E form typical colonies. Under serum-deprived culture conditions the combination of IL-3 and GM-CSF results in more BFU-E than either factor alone when EPO is added on day 3. Although EPO is crucial for the terminal differentiation of erythroid progenitors, mice with homozygous null mutations of the Epo or Epor genes form BFU-E and CFU-E normally, but they fail to differentiate into mature erythrocytes. Both the Epo −/− and Epor −/− mutations are embryonically lethal due to failure of definitive (fetal liver) erythropoiesis. However, yolk sac erythropoiesis is only partially impaired, indicating that a population of EPO-independent primary erythropoietic precursors exists. SCF has marked synergistic effects on BFU-E cultured in the presence of EPO, although alone it has no colony-forming ability. SCF is crucial for the normal development of CFU-E, because mice that lack SCF (Steel mutants) or its receptor Kit (W mutants) are severely anemic and show a reduction in fetal liver CFU-E. Further studies showed that SCF induces tyrosine phosphorylation of the EPOR and that Kit associates with the cytoplasmic domain of the tyrosine phosphorylated EPOR. Further insight into the molecular mechanism by which Epo prevents apoptosis in erythroid cells comes from studies of Stat5a −/− 5b −/− mice. During fetal development the embryos are severely anemic; the erythroid progenitors in fetal liver are reduced in number and show increased apoptosis. This result was explained by the finding that STAT5 mediates the early induction of the antiapoptotic gene Bcl-X L through direct binding to its promoter. Interestingly, although adult animals were thought to be hematologically normal, about half have chronic anemia with splenomegaly, due to an increase in early erythroid precursors; erythropoiesis is ineffective because of an increase in apoptosis in these early normoblasts that show reduced levels of Bcl-X L .
Factors distinct from the classic CSFs may positively regulate erythropoiesis, either directly or indirectly. Limiting-dilution studies of highly purified CFU-E in serum-free culture show that insulin and insulin-like growth factor 1 (IGF-1) act directly on these cells. The presence of Epo is essential in these studies, which contrast with earlier murine studies of unfractionated cells in which CFU-E respond to IGF-1 or insulin in the absence of Epo. Activin is another factor that enhances both BFU-E and CFU-E colony formation. This protein dimer, also known as follicle-stimulating hormone–releasing protein, appears to have a lineage-specific effect on erythropoiesis that is indirect because removal of monocytes and/or T lymphocytes abrogates its effect. It is interesting that activin has been identified as the factor produced by vegetal cells during blastogenesis that induces animal ectodermal cells to form primary mesoderm. Hepatocyte growth factor has also been shown to have synergistic effects on CFUs-GEMM and BFU-E in cultures containing EPO.
Negative Regulation of Erythropoiesis
Observations that subsets of lymphocytes with an immunologic suppressor phenotype isolated from normal subjects can inhibit erythroid activity in vitro correlate with reports of patients with a variety of disorders in whom anemia or granulocytopenia is associated with an expansion of certain T-lymphocyte populations (see also Chapter 7 ). In the rare disorder T lymphocytosis with cytopenia, in vitro suppression of erythropoiesis has been correlated with the expansion of a T-lymphocyte population that may be the counterpart of the hematopoietic suppressor cells isolated from normal blood. The phenotype of these cells has been described in detail. The cell is a large granular lymphocyte that is positive for both E rosettes and CD8 (classic suppressor phenotype). Suppressor T cells may also be involved in some cases of aplastic anemia or neutropenia without an underlying immunologic disorder or an overt T-cell proliferation. Exactly how such suppressor T cells interact with hematopoietic progenitors and what surface antigens are “seen” by the suppressors is uncertain. There is evidence to support the concept that suppression of erythroid colony expression in vitro is regulated by T cells and may be genetically restricted. Cell-cell interactions in immunologic systems have been well characterized with regard to surface determinants that allow for cellular recognition. That certain phenotypes of T cells “recognize” distinct classes of histocompatibility antigens on immunologic cell surfaces has been well described. Thus the observation that hematopoietic progenitors have a unique distribution of class II hiscompatibility antigens on their cell surfaces suggests a role for these antigens in the cell-cell interactions that regulate hematopoietic differentiation.
TNF also suppresses erythropoiesis in vitro. The injection of peritoneal macrophages into Friend murine leukemia virus–infected animals results in rapid but transient resolution of the massive erythroid hyperplasia associated with this disease. This may be due to elaboration by macrophages of IL-1α, which does not suppress erythropoiesis itself but acts by the induction of TNF. This effect is reversed by Epo.
Myelopoiesis
Phagocyte Development
Although a clonogenic assay of granulocyte progenitors was developed almost a decade before the erythroid clonogenic assay, the various factors responsible for granulocyte-macrophage development remain incompletely understood. Excellent reviews of early work in this field have been provided by Metcalf and Moore.
It is now accepted that the granulocyte-macrophage colony-forming unit, or CFU-GM, is derived from the pluripotent progenitor and, under the influence of SCF, IL-3, GM-CSF, G-CSF, and/or M-CSF, ultimately gives rise to mature granulocytes and monocytes. Monocytes irreversibly leave the circulation and differentiate further into fixed-tissue macrophages, a category comprising the alveolar macrophages, hepatic Kupffer cells, dermal Langerhans cells, osteoclasts, peritoneal and pleural macrophages, and, perhaps, brain microglial cells. The enormous diversity of this system and the high turnover rate of granulocytes, as well as the necessity of maintaining splenic, marginated, and bone marrow granulocyte pools in order to meet sudden demands caused by infection, have led to the evolution of an extremely complex regulatory network. Granulopoiesis associated with myelosuppression, cyclic neutropenia, and infection depends on IL-23 production by macrophages and dendritic cells that in turn stimulates IL-17 and G-CSF upregulation, a pathway revealed by study of leukocyte adhesion molecule–deficient mice. Recent data suggest that the innate immune mechanisms that involve Toll-like receptor 4 (TLR4) and its adaptor protein TRIF may be important in steady-state and emergency granulopoiesis.
Transcription Factors and Myelopoiesis
A family of transcription factors important for commitment to myeloid and lymphoid lineages as well as regulation of myeloid-specific promoters such as those of the myeloperoxidase (MPO) and neutrophil elastase (NE) genes are factors that were first identified on the basis of their ability to bind to the polyomavirus enhancer–binding protein 2/core binding factor (PEBP2/CBF). Two alpha subunits (CBFα1 and CBFα2 [the human homologue is known as AML-1 or RUNX1 ]) bind DNA with low affinity, and affinity is strengthened in the presence of the non–DNA-binding beta subunit (CBFβ). As noted above, runx1 −/− mice die in utero by day 12.5 because of failure of production of all definitive hematopoietic lineages. The CCAAT/enhancer-binding protein (C/EBP) family of transcription factors bind to DNA through a basic region–leucine zipper domain (bZIP). There are several family members (C/EBPα, C/EBPβ (NF–IL-6), C/EBPγ, C/EBPδ, C/EBPζ, and C/EBPε) that are differentially expressed during myelopoiesis, with increasing (C/EBPβ), or an increase followed by either a partial (C/EBPδ) or a marked (C/EBPα) reduction in level of, expression during maturation of 32D cl3 cells to terminally differentiated granulocytes. C/EBPα has been implicated in the regulation of hepatocyte and adipocyte differentiation (levels are low in undifferentiated dividing cells but high in quiescent terminally differentiated cells), and also in myeloid CSF receptor promoter function; RUNX1 can act synergistically with C/EBPα to activate the M-CSFR promoter. C/ebp α −/− mice show arrested myelopoiesis at the myeloblast stage resulting from failure of expression of G-CSF and IL-6 receptors. Mice that lack C/EBPβ produce monocytes that are defective in bactericidal and tumoricidal function; their macrophages and fibroblasts, but interestingly not their endothelial cells, fail to produce G-CSF in response to LPS. Thus C/EBPβ is important for “emergency” granulopoiesis. C/EBPε is produced primarily in myeloid lineages. Knockout mice are viable and fertile but die at 50 to 75 days from opportunistic infections with Pseudomonas aeruginosa . Nullizygous animals fail to produce normal neutrophils and eosinophils. Functional abnormalities include diminished release of hydrogen peroxide in response to phorbol myristate acetate ( PMA) stimulation, because of failure to produce secondary and tertiary granules, which are important reservoirs of membrane-bound components of the nicotinamide adenine dinucleotide phosphate (NADPH) oxidase apparatus. Additional components of secondary and tertiary granules, lactoferrin and gelatinase B, are absent because of failure of transcriptional activation of C/EBPε. Interestingly, similar abnormalities characterize the rare neutrophil-specific granule deficiency, and indeed mutations in C/EBP ε have recently been found in these patients. The PU.1(Spi-1) transcription factor is a member of the Ets family and is expressed principally in monocytes/macrophages and B lymphocytes, and also in erythroid cells and granulocytes. Potential target genes include the integrin CD11b, M-CSFR, GM-CSFRα, G-CSFR, and the Igλ light chain. Mice that lack PU.1 die in utero with absence of monocytes, granulocytes, and T and B lymphocytes; anemia is variable and thus does not explain the prenatal mortality.
Growth Factors and Myelopoiesis
Murine IL-3 stimulates a broad spectrum of myeloid progenitor cells, including pluripotent stem cells, granulocyte and/or macrophage colony-forming cells or units (CFU-GM, CFU-G, and CFU-M), erythroid burst-forming units (BFU-E), eosinophil colony-forming units (CFU-Eo), megakaryocyte colony-forming units (CFUs-Meg), and mast cells. As its name implies, GM-CSF was initially shown to be more restricted as a stimulus of the proliferation and development of CFU-GM. However, murine studies with purified or recombinant factor have shown that it stimulates the initial proliferation of other progenitors such as BFU-E as well (see above). The other murine factors, G-CSF and M-CSF, are more restricted and predominantly stimulate granulocyte and monocyte colony-forming units (CFU-G and CFU-M), respectively.
With the possible exception of GM-CSF, the activities of the human CSFs are similar to those of the corresponding murine factors. Both IL-3 and GM-CSF affect a similar broad spectrum of human progenitor cells. This includes CFU-GEMM, CFU-GM, CFU-G, CFU-M, CFU-Eo, and CFU-Meg. In full-serum cultures, IL-3 and GM-CSF alone stimulate the formation of colonies derived from CFU-GM, CFU-G, CFU-M, CFU-Eo, and CFU-Meg. Data from serum-free cultures suggest that in the presence of IL-3 or GM-CSF alone, myeloid colony formation is much reduced, and that optimal CFU-G or CFU-M proliferation requires the addition of G-CSF or M-CSF, respectively, to the cultures. Even in serum-replete conditions, IL-3 acts additively or synergistically with G-CSF to induce more granulocyte colony formation than is observed with either factor alone.
Insight into the in vivo role of GM-CSF comes from studies in which the GM-CSF gene was disrupted by homologous recombination in embryonic stem cells. GM-CSF knockout mice showed normal basal hematopoiesis but developed progressive accumulation of surfactant lipids and proteins in the alveolar space, the defining characteristic of idiopathic human pulmonary alveolar proteinosis. Extensive lymphoid hyperplasia associated with lung airways and blood vessels was also found. Surfactant proteins and lipids are synthesized by type II pneumocytes and cleared from the alveolar space by type II cells and by alveolar macrophages. The lungs from the null animals showed normal surfactant synthetic capacity and no accumulation in type II pneumocytes. In contrast, the alveolar macrophages showed a marked increase of surfactant protein and lipid, suggesting strongly that these cells cannot process surfactant as a result of the lack of GM-CSF. Mice that were generated with null mutations of the common chain of the GM-CSF/IL-3/IL-5 receptor (βc) show similar pulmonary pathology, and also show low basal numbers of eosinophils and absence of blood and lung eosinophilia in response to infection with the parasite Nippostrongylus brasiliensis . The G-CSF gene has also been disrupted by homologous recombination in embryonic stem cells. G-CSF −/− mice have a chronic neutropenia (20% to 30% of normal levels) and reduced bone marrow myeloid precursors and progenitors. The animals also had a markedly impaired capacity to increase neutrophil and monocyte counts after infection with Listeria monocytogenes .
In addition to their effects on progenitor differentiation, the CSFs also induce a variety of functional changes in mature cells. GM-CSF inhibits polymorphonuclear neutrophil migration under agarose, induces antibody-dependent cytotoxicity (ADCC) for human target cells, and increases neutrophil phagocytic activity. Some of these functional changes may be related to GM-CSF–induced increase in the cell surface expression of a family of antigens that function as cell adhesion molecules. The increase in antigen expression is rapid and is associated with increased aggregation of neutrophils; both are maximal at the migration inhibitory concentration of 500 pM, and granulocyte-granulocyte adhesion can be inhibited by an antigen-specific monoclonal antibody. GM-CSF also acts as a potent stimulus of eosinophil ADCC, superoxide production, and phagocytosis. G-CSF acts as a potent stimulus of neutrophil superoxide production, ADCC, and phagocytosis, whereas M-CSF activates mature macrophages and enhances macrophage cytotoxicity.
Megakaryocytopoiesis
The cloning of thrombopoietin (TPO) has greatly clarified our understanding of the regulation of megakaryocytopoiesis. Prior to the discovery of TPO, several factors, including IL-3, IL-6, IL-11, SCF, and even erythropoietin were shown to stimulate megakaryocytopoiesis and thrombopoiesis in vitro and in vivo. IL-3, IL-6, and IL-11 engage heterodimeric receptors of the beta common family (IL-3R) and gp130 family (IL-6R and IL-11R). SCF engages a receptor the intracellular domain of which expresses tyrosine kinase activity upon ligand binding. As already emphasized in this chapter, ligand engagements of these receptor families are known to induce early multipotent progenitors to proliferate and even differentiate toward lineage-specific progenitors, and SCF, IL-3, and IL-11 participate in the induction of the proliferation and differentiation of lineage-specific progenitors. Single-cell analysis of prospectively isolated murine megakaryocyte progenitors shows that a large proportion (≈60%) express EPOR, which is consistent with the potent effect of EPO on megakaryocyte colony formation.
The discovery of TPO provides a major step in understanding megakaryocytopoiesis because this factor induces lineage-restricted megakaryocyte progenitor proliferation, differentiation of those committed progenitors to megakaryoblasts, and, finally, differentiation of megakaryoblasts to the megakaryocytes that in turn produce platelets.
Thrombopoietin
TPO is produced at the site of hematopoiesis. Therefore, though its activity is increased in the blood during episodes of thrombopenia, it does not necessarily function as a hormone, because it is produced directly at the site of thrombopoiesis. In this sense, it differs from erythropoietin, which is not produced at all in marrow stroma. Blood levels may increase in thrombopenic states merely because circulating platelets and/or tissue megakaryocytes sop up the growth factor and carry it out of the circulation. This theory has received support from observations in mice with disruption of the murine transcription factor gene called NF-E2 ; although these animals are thrombocytopenic they have an increase in megakaryocyte mass and no increase in serum TPO levels.
As reviewed by Kaushansky, the TPO molecule is considerably longer than the other HGF polypeptides. Its 5′ half bears 23% sequence homology to erythropoietin, whereas the 3′ half bears no structural homology to any cytokine and may be removed by a proteolytic mechanism. Indeed, removal of this half does not ablate physiologic function. The resemblance of the 5′ domain of the molecule to erythropoietin may explain the synergy of thrombopoietin and erythropoietin in megakaryocyte colony formation and platelet production. It is well-recognized that splenectomized individuals with persistent anemia usually have significant thrombocytosis and many individuals with red cell aplasia and high EPO levels also have thrombocytosis and megakaryocytosis.
The function of TPO has been studied carefully in vivo and in vitro. Lineage-specific CD34+ meg progenitors bear receptors for SF, IL-3, IL-11, and TPO, the four major classes of hematopoietic cytokine receptors. Maximal megakaryocyte colony formation probably requires signaling by all four receptors, but TPO is absolutely required for the final stages of CFU-MEG maturation, including maximal ploidy and cytoplasmic volume, and, therefore, platelet production. Therapeutic trials of TPO in mice have shown that TPO is species specific. Treatment of mice with murine TPO induces massive thrombocytosis, whereas human TPO is much less active in these animals. More importantly, TPO is active in reducing the platelet nadir in mice and primates rendered thrombopenic by chemotherapy or irradiation.
Circulating Platelets
The differential diagnosis of thrombocytopenia rests first on evaluation of platelet morphology. In conditions in which megakaryocytopoiesis is accelerated, circulating platelet volume (and usually diameter) is increased. The reasons for this shift in volume are disputed. Some claim that young platelets are larger than old platelets. Others suggest that large megakaryocytes give rise to large platelets. Neither explanation satisfies all experimental and clinical conditions, but in general thrombocytopenia secondary to increased destruction of platelets is associated with platelets of large volume, and thrombocytopenia related to decreased production of platelets is associated with platelets of normal size. There are major exceptions to this rule. Patients with hyposplenism tend to have large platelets in their blood, whether thrombopoiesis is increased or not, and patients with primary abnormalities of platelet function, such as Wiskott-Aldrich syndrome or Bernard-Soulier syndrome, have platelet sizes that bear no relationship to platelet production. Thrombopoietin increases platelet production by increasing both the number and size of individual megakaryocytes. Though TPO is probably solely responsible for the later stages of recognizable megakaryocyte differentiation and proliferation of megakaryocyte progenitors, its function depends, at least in part, on the additional stimulation of megakaryocyte progenitors (and probably early megakaryocytes as well) with other growth factors, including IL-3, IL-11, SF, and EPO.
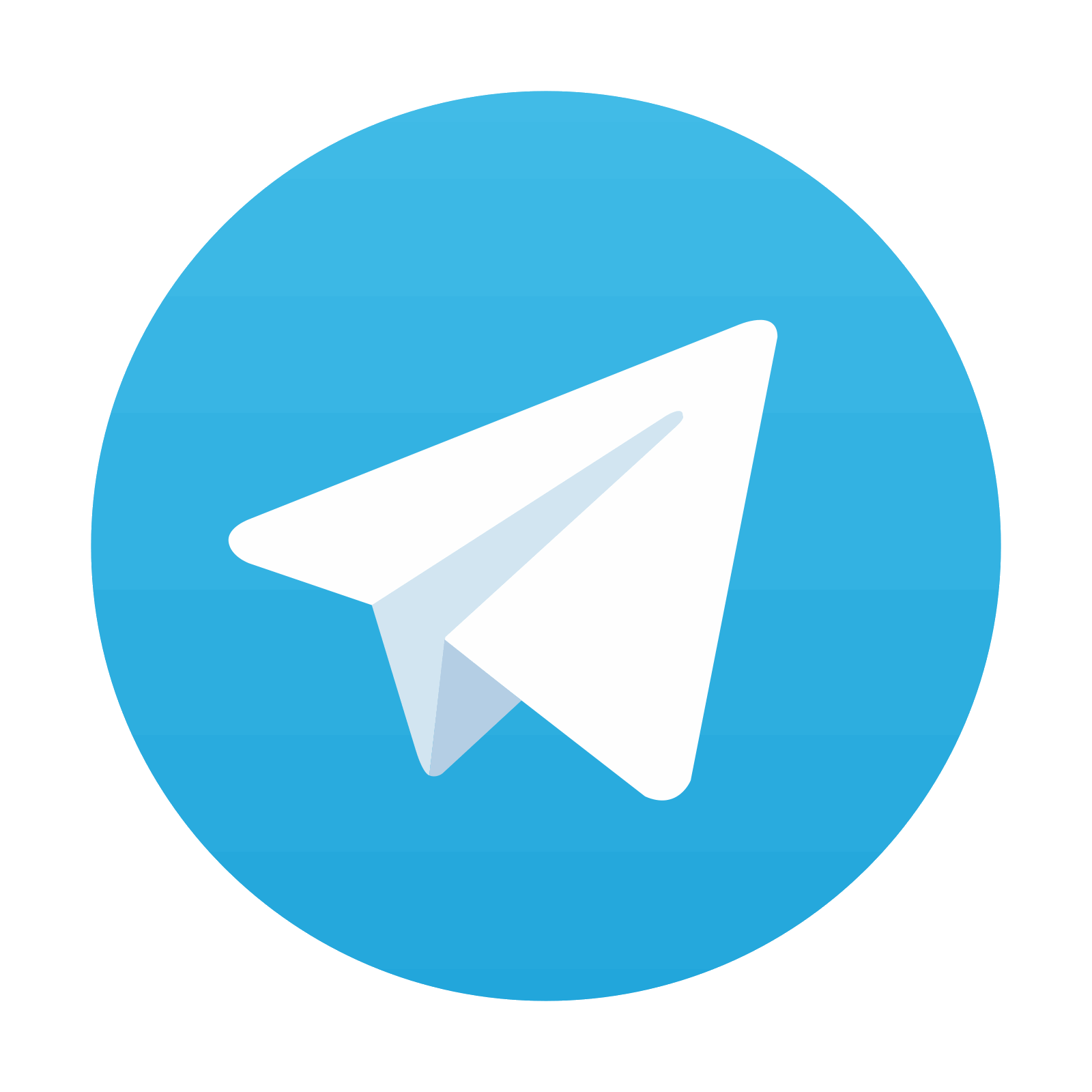
Stay updated, free articles. Join our Telegram channel
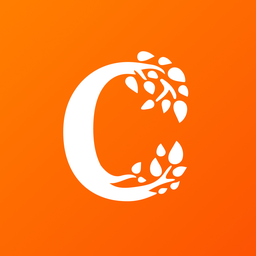
Full access? Get Clinical Tree
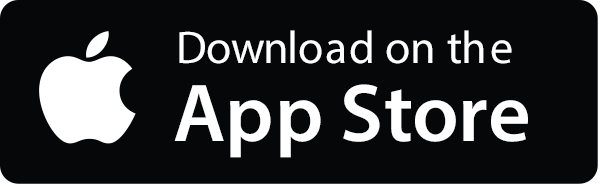
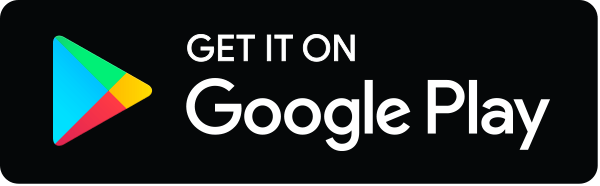