Acquired
Malignant
Nonmalignant
Congenital
Acute nonlymphoblastic
Leukemia
Acute lymphoblastic leukemia
Chronic myelogenous leukemia
Chronic lymphocytic leukemia
Non-Hodgkin lymphoma
Hodgkin disease
Multiple myeloma
Myelodysplastic Syndrome
Myelofibrosis
Agnogenic myeloid metaplasia
P. vera
Ess. thrombocythemia
Myeloproliferative disorders
Neuroblastoma
Hypereosinophilic syndrome
Carcinoma of the breast
Ovarian carcinoma
Renal cell carcinoma
Other selected solid tumors
Aplastic anemia
Pure red cell aplasia
Paroxysmal nocturnal hemoglobinuria
Acquired immunodeficiency
Syndrome
Autoimmune disorders
Systemic sclerosis
Systemic lupus erythematosus
Rheumatoid arthritis
Multiple sclerosis
Immunodeficiencies
Severe combined
immunodeficiencies
Combined immunodeficiencies
Leukocyte adhesion defects
Actin deficiency
Chronic mucocutaneous
candidiasis
Others
Hematologic defects
Wiscott–Aldrich syndrome
Fanconi anemia
Blackfan–Diamond anemia
Thalassemia
Sickle cell disease
Glanzmann’s thrombasthenia
Gaucher’s disease
Chronic granulomatous disease
Congenital neutropenia
Chediak-Higashi syndrome
Langerhans cell histiocytosis
Dyskeratosis congenita
Congenital amegakaryocytosis
Thrombocytopenia-absent-radius (TAR) syndrome
Familial erythrophagocytic lymphohistiocytosis (FEL)
Others
Osteopetrosis
Mucopolysaccharidases
Hurler syndrome
Hunter syndrome
Maroteaux–Lamy syndrome
Others
Mucolipidoses
Metachromatic leukodystrophy
Adrenoleukodystrophy
Other lipidoses
Other lysosomal diseases
Lesch–Nyhan syndrome
Type IIa glycogen storage
Disease
Depending upon the rationale for the use of HSC, they may be collected from the patients’ themselves (autologous) or collected from a donor other than the patient (allogeneic), or, in the rare case of an identical twin, (syngeneic).
Autologous HSCT is currently utilized primarily as a rescue strategy to overcome dose-limiting hematopoietic toxicity of chemoradiotherapy. Autologous transplantation is used to treat several malignancies in which dose intensification of therapy to myeloablative levels is potentially curative or capable of delaying disease recurrence. For patients undergoing autologous HSCT to treat autoimmune disorders, the rationale is that intensive conditioning eradicates autoimmune cells and may allow a new and healthy repertoire of immune cells to regenerate and selectively increase regulatory T cells [29]. Active investigation of the potential of gene therapy to treat primary immune deficiencies, hemoglobinopathies, and metabolic diseases is ongoing. Additional information regarding clinical data on autologous transplantation is presented at the conclusion of this chapter.
Allogeneic HSCT, in contrast to just the rescue properties of autologous or syngeneic HSCT, can be additionally curative of a malignancy via a GVT effect [30]. This effect, which is mediated by donor lymphocytes, results in donor cell-mediated destruction of residual disease. Recognition of the GVT effect has led investigators to develop minimally toxic yet adequately immunosuppressive preparative conditioning regimens to facilitate engraftment of donor cells and allow subsequent GVT effects to eradicate the malignancy. These regimens, which are discussed in more detail in the next section, can be administered even to older individuals or patients with comorbid conditions who cannot withstand the toxicity that may be associated with high-intensity conditioning regimens. However, because a GVT effect may take time to develop and it is unlikely to have the capacity to overcome aggressive, active disease, these regimens are most effective in patients with indolent diseases or patients in complete remission (CR) at the time of transplantation [31–33]. For younger, fitter patients, and patients at higher risk of relapse, high-intensity conditioning is generally still desired prior to allogeneic HSCT to minimize disease burden and decrease relapse risk.
Allogeneic HSCT can also be used as a curative strategy for nonmalignant congenital or acquired conditions that result in ineffective hematopoiesis, immunodeficiencies, or certain metabolic disorders. The allograft corrects the deficiency and reconstitutes a normal hematopoietic system. Here, the goal is to minimize regimen-related toxicity and avoid GVHD. Because these patients often have not been treated with cytotoxic, immunosuppressive therapies prior to HSCT, their immune systems are frequently more intact, and securing engraftment can be more challenging [34, 35].
Preparation for Transplantation
High-Intensity Regimens
These intensive regimens ablate the patient’s marrow and if not salvaged with HSC infusion would result in aplasia or severely prolonged cytopenias. High-intensity preparative regimens include chemotherapeutic agents such as busulfan, etoposide, melphalan, thiotepa, cytarabine, carmustine, and are often combined with irradiation, either in the form of TBI, limited field irradiation, or targeted radioimmunotherapy.
Reduced-Intensity Conditioning Regimens
Broadly, these regimens are designed to be less toxic than high-intensity regimens, but they represent a spectrum of intensity. The most minimally toxic regimens are designed to provide only sufficient immunosuppression to facilitate engraftment of donor cells. Mixed chimerism between donor and host cells frequently develops for a period following transplantation. For nonmalignant disorders, mixed chimerism may be sufficient to correct the underlying condition, but to treat malignancies, complete donor chimerism is generally desired as donor cells that are not tolerant of host antigens are required to mediate destruction of residual disease.
Two gray (Gy) TBI ± 90 mg/m2 of fludarabine, pioneered in Seattle, is the paradigmatic low-intensity regimen for patients undergoing HSCT for malignant diseases [36]. However, for patients undergoing transplantation for nonmalignant conditions, or those who have not received significant prior therapy for their malignancies, additional immunosuppressive therapy may be required to secure engraftment and avoid graft rejection. Modest increases in radiation doses, or added T-cell-specific therapies such as antithymocyte globulin (ATG) or alemtuzumab (anti-CD52 antibody), may be incorporated into these regimens [37, 38].
Additionally, because relapse remains a significant issue following reduced-intensity conditioning (RIC) transplants, several regimens have been developed that are designed to be less toxic than traditional high-intensity regimens, but potent enough in their antimalignant capacity to reduce disease burden sufficiently to allow GVT effects to mediate host cell elimination. Fludarabine combined with modest doses of busulfan, cyclophosphamide, melphalan, or TBI is typically used in those regimens [39–42]. Treosulfan, a busulfan analogue with less liver toxicity and possibly greater antileukemic potential, is currently under investigation as a novel agent in RIC regimens, and initial data suggest lower toxicity [43, 44]. Targeted radioimmunotherapy has also emerged as a strategy to intensify radiation therapy with minimal toxicity. Radiolabeled antibodies targeting receptors expressed on hematopoietic elements allow focal intense radiation exposure to disease with minimal exposure to other organs. Radiolabeled CD20 antibodies have been well established in the treatment of lymphoma, and radiolabeled CD45 antibody shows promising early data in the treatment of myeloid leukemia and myelodysplastic syndrome (MDS) [43, 45]. New strategies to pretarget radiolabeled antibodies directly to malignancy may lead to even greater efficacy of this strategy [46].
Selection of Hematopoietic Stem Cell Donors
Three questions are addressed when deciding upon an HSCT donor. First, will the transplant be autologous or allogeneic? Second, for allogeneic transplantation, will the donor be related or unrelated, or will cord blood be used? Third, will the stem cells be collected from the bone marrow or peripheral blood or cord blood? Characteristics of these stem cell sources are summarized in Tables 57.2 and 57.3.
Table 57.2
Hemopoietic stem cell sources
Source | Indication |
---|---|
Marrow/peripheral blood | |
HSC harvested from peripheral blood originate from marrow and recirculate to the marrow. However, their differentiation status may differ from that of marrow HSC, and they are further modified by exposure to G-CSF which “mobilizes” them from the marrow. PBSC lead to faster hematopoietic recovery post-transplant than cells aspirated from unmanipulated marrow. Also, PBSC can possibly be obtained even if aspiration from marrow is not possible. | |
Allogeneica | Acquired diseases with or without HSC involvement; congenital disorders. |
Syngeneic | Acquired malignant or nonmalignant diseases with or without HSC involvement. |
Autologous | Acquired, usually malignant disorders, generally without HSC involvement; autoimmune disorders; possibility of genetic correction of defect. |
Umbilical cord blood | |
Allogeneica | Lack of alternative stem cell source; acquired diseases with or without HSC involvement; congenital disorders. |
Autologous | Experimental; possibility of genetic correction of defect. |
Table 57.3
Characteristics of marrow, peripheral blood, and cord blood used for allogeneic HSCT
Characteristic | Marrowb | Peripheral blood | Cord blood |
---|---|---|---|
Harvest procedure | Anesthesia—Multiple marrow aspirates | Vascular access—Apheresis (» 4 h × 1 – 2)a | Extraction of cells from cord and placenta |
Number of cells obtained (per kg patient weight) | |||
•Total mononuclear | 1 – 4 × 108 | 2 – 100 × 108 | 0.7 – 30 × 107 |
•CD34+ | 1 – 5 × 106 | 2 – 20 × 106 | <1 – 45 × 105 |
•T cells | 5 – 50 × 106 | 2 – 10 × 108 | <5 – >20 × 106 |
Cell subpopulations | |||
•CD34+ | c | ↑ # | ↑ # |
•T cells | c | ↑ #; ↓ reactivity | ↓ Reactivity |
•Monocytes | c | Increased IL-10 | Reduced HLA-DR, CD86, ICAM1, IFN-γ production |
Autologous vs. Allogeneic Transplantation
As described earlier, autologous transplantation is indicated for several diseases. While autologous HSC in principle should be available for every patient (except possibly heavily pretreated patients or patients with marrow failure states who are unable to mobilize sufficient stem cells for subsequent transplantation), autologous transplantation conveys no GVT effect and there is always concern that some malignant (diseased) cells are returned to the patient with the transplant inoculum. Until more effective gene transfer strategies have been developed, autologous HSC treatments are also not widely available for genetically determined disorders. Given its inherent risks, allogeneic transplantation is generally reserved for diseases not amenable to treatment with autologous transplantation. Allogeneic transplantation is virtually always performed with curative intent.
Allogeneic Donor Selection
HLA matching is the primary consideration when selecting an allogeneic donor [47]. The Class I molecules HLA-A, B, C and the Class II molecules HLA-DR ± DQ are considered in screening potential donors. In general, an HLA-matched sibling donor is considered the first choice. Because individuals inherit one set of HLA genes from each parent, any sibling has a 25% chance of being matched to the patient, and ∼30% of persons in the United States will have an HLA-matched sibling [48]. In addition, phenotypically-matched related donors are identified for about 1% of patients, and somewhat less than 1% of patients will have an identical (syngeneic) twin donor [49]. Syngeneic donors, however, do not provide a GVT effect.
For patients lacking a matched sibling, an alternative donor must be identified. The typical second choice is an unrelated donor matched at high-resolution (DNA level) typing for at least 8–10 HLA antigens. Due to the efforts of organizations such as the Anthony Nolan Appeal in the UK, the National Marrow Donor Program in the United States, and other organizations worldwide, more than 12 million volunteer donors have been added to registries as potential HSCT donors [50]. Computer searches of those databases very quickly generate an idea as to the probability of finding an unrelated donor. However, the logistics of confirming eligibility and coordinating timing of an unrelated donor frequently take weeks to months, and this delay may be too long to control disease in patients with aggressive malignancies [51]. The probability of finding a “complete” HLA match is currently about 50–60% for Caucasian patients. The probabilities are lower for patients of different ethnic backgrounds [48].
For patients lacking a matched unrelated donor, alternative donor options include mismatched unrelated donors, UCB, and haploidentical donors. UCB, due to its unique immunologic qualities discussed later, has less stringent matching requirements than unrelated donors, and an acceptable cord blood match is therefore more easily attainable. A significant body of rapidly increasing data supports the efficacy of UCB transplantation for patients lacking suitably-matched related or unrelated donors. Haploidentical donors—related donors who share only one haplotype (the maternal or paternal #6 chromosome) with the patient—are appealing, because nearly all patients will have a donor who is immediately available (at low search cost). However, the high degree of HLA mismatching inherent to haploidentical transplantation creates significant immunologic challenges, including potential graft rejection, GVHD, and poor immune reconstitution, and haploidentical transplantation at present remains investigational.
The Source of Hematopoietic Stem Cells
Once a donor is identified, a final consideration is whether to use HSC collected from bone marrow or peripheral blood; in some cases, cord blood might be the first choice. Methods of collecting and infusing HSC from these various sources are discussed in the subsequent section.
Bone Marrow vs. Peripheral Blood
Bone marrow was the original source of HSC evaluated for transplantation. Demonstration of the ability to mobilize HSC into the peripheral blood either with high-dose granulocyte colony-stimulating factor (G-CSF) or chemotherapy ± glucocorticoids followed by G-CSF raised the possibility of peripheral blood stem cells (PBSC) as a source for harvesting cells [52–54]. PBSC show a lower retention of Rhodamine 123 (which is typical for noncycling cells), and may have a lower expression of CD38 as compared to bone marrow cells. Unless T cell depleted, PBSC contain significantly greater numbers of T cells than marrow.
An initial concern with PBSC was that they may not be capable of long-term hematopoietic reconstitution. These concerns have been definitively rebuked based on results from autologous transplant patients who have been followed now for more than 15 years and show normal hematopoiesis, as well as observations with allogeneic PBSC transplants, which have resulted in sustained donor cell engraftment [55, 56]. Given the ease of collecting PBSC relative to marrow, the number of PBSC transplants as compared to marrow transplants has increased significantly, and PBSC are currently used for virtually all autologous stem cell transplants in patients with hematologic malignancies. Several issues regarding the relative benefits of PBSC vs. bone marrow, however, are worthy of discussion.
Numerous randomized trials have compared outcomes using either bone marrow or PBSC for patients undergoing allogeneic HSCT following high-intensity conditioning for hematologic malignancies. A meta-analysis reviewing nine trials and 1,288 patients explored the relative benefits of each stem cell source [57]. Engraftment (neutrophils ≥0.5 × 109/l) was significantly more rapid following PBSC (median 14 vs. 22 days p < 0.00001). (Of note, G-CSF mobilization of bone marrow prior to bone marrow collection may decrease time to engraftment of cells harvested from the bone marrow [58].) There was no difference between overall rates of acute GVHD between donor sources, but there was a modestly higher incidence of grades III–IV acute GVHD following PBSC transplantations (p = 0.03). Patients treated with PBSC had a higher incidence of extensive chronic GVHD (p < 0.00001). Rates of relapse were lower among PBSC patients, and PBSC were associated with improved disease-free survival, particularly among patients with more advanced disease at the time of transplantation. There was no statistically significant difference in overall survival between patients, but the subgroup of patients with advanced disease had improved survival following the use of PBSC. In the aggregate, a recent analysis suggests that for patients with a 1 year relapse risk > 5%, PBSC are preferable to marrow both in terms of overall and quality-adjusted life expectancy [59].
In patients who are prepared for transplantation with RIC regimens, PBSC have been used virtually exclusively as the source of HSC. This approach has been taken in part because of the greater “engraftment potential” ascribed to those cells and in part related to the larger numbers of cells that are obtainable and the higher T-cell doses [57].
However, in patients undergoing HSCT for nonmalignant disorders, concern about the potential increased risk of GVHD associated with PBSC still favors the use of marrow [57]. Patients with nonmalignant diseases are unlikely to derive any gain from the development of chronic GVHD. Similarly, for patients undergoing haploidentical transplantation, PBSC have been avoided in favor of bone marrow or CD34+-selected products, given the high risk of GVHD.
Cord Blood
Cord blood has emerged as an important source of HSC. Since the first UCB transplant was successfully performed in 1988 on a 5-year-old child with Fanconi anemia using his HLA-identical sibling’s UCB [60], rapid developments in the field have led to a dramatic increase in the use of UCB. Studies demonstrate that the proliferative potential of cord blood stem cells, as determined by the generation of CD34+ cells in serum-free medium, is greater than that of adult marrow or PBSC [61]. Cord blood contains proportionally higher numbers of progenitor cells per volume than adult blood and possibly adult marrow [17, 62, 63]. Immunological studies show that cord blood T cells are less immunocompetent than adult cells [60, 63]. Cord blood T cells can be activated via the T-cell receptor, but have a reduced capacity to stimulate [64]. Intracytoplasmatic signaling (following T-cell receptor engagement) in cord blood T cells also differs from that in adult T cells [65]. Cord blood monocytes express lower levels of HLA-DR, CD86, and ICAM-1 than monocytes in adult blood, and produce lower levels of IL-10 and IFN-γ. IL-4 and IL-5 are basically absent [64]. These differences in the immunologic characteristics of UCB may contribute to the tolerability of greater mismatching between donor and patient. Additional advantages of cord blood include the fact that it is a frozen off-the-shelf product that is rapidly available, that there is no danger to the donor, and that the risk of transfer of viruses is minimal. Major challenges to using UCB include the low number of HSC and progenitors in a single cord unit, which can lead to delayed engraftment and graft failure—particularly in adults and large children—as well as possible delays in immune reconstitution.
Harvesting and Infusing HSC for Transplantation
Bone Marrow
In an adult, bone marrow is the primary organ of hematopoiesis and the site from which stem cells for transplantation were traditionally harvested [66]. For the purpose of marrow harvest, the donor (or patient) receives anesthesia, and under sterile conditions, multiple small volume aspirates (≈3 ml) of marrow are obtained from both posterior iliac crests [67]. Additional potential aspiration sites are the anterior iliac crests, the sternum, and, particularly in children, the tibia heads. Approximately 10–15 ml/kg donor weight is collected, usually yielding 1–4 × 108 cells/kg recipient weight (Table 57.4). The concentration of cells is generally higher in children than in adults, and in first harvests than in subsequent aspirations if done within a short time interval after the first procedure [68–70]. The marrow is passed through filters, generally in semiclosed systems, to break up cell aggregates and remove bone particles. If no ABO incompatibility exists and if the marrow is not to be subjected to any in vitro purging procedure or other manipulation, the resulting cell suspension is infused intravenously, generally via an indwelling intravenous catheter (e.g., Hickman line), and cells “home” through the peripheral blood circulation to the marrow cavity to reconstitute the hematopoietic system.
Table 57.4
Autologous and allogeneic peripheral blood stem cell (precursor) harvesting
HSC source | ||
---|---|---|
Autologous | Allogeneic | |
Methods of mobilization | Chemotherapy, cytokines/growth factors (antibodies to adhesion molecules) | Cytokines/growth factors |
Types of chemotherapy | Cyclophosphamide; etoposide; paclitaxel; epirubicin; ifosfamide; cisplatin; carboplatin (combinations)a | – |
Cytokines/growth factors used | G-CSF (5–20 μg/kg/day) GM-CSF (4–64 μg/kg; 125–250 μg/m2) IL-3 (5–10 μg/kg) TPO/MGDF (0.03–5 μg/kg) SCF (10–25 μg/kg) (combinations)b | G-CSF (2–20 μg/kg/day) |
Risk of tumor cell contamination | Yes (higher without chemotherapy) | No |
Time course for harvest | Predictable for cytokines; variable for chemotherapy | Predictable |
Peripheral Blood Stem Cells
At steady state, HSC circulate at very low concentrations in peripheral blood. Following cytotoxic therapy, however, the frequency of early hematopoietic precursors in the circulation increases dramatically, though transiently, during the recovery phase [52]. The same effect is achieved if cytotoxic agents such as cyclophosphamide, etoposide, or taxol are given for the very purpose of “mobilizing” cells from the marrow into the circulation (Table 57.4) [71]. The administration of recombinant hematopoietic growth factors, such as G-CSF, granulocyte-macrophage colony-stimulating factor (GM-CSF) or c-kit ligand alone or in combination, has similar effects (although presumably via different mechanisms [72]), and the timing of the maximum stem cell yield, around 4–6 days after initiation of treatment with growth factors, is more predictable than with chemotherapy [53, 54]. Furthermore, the absence of cytotoxicity and the associated side effects allows the application of this approach to normal donors and the harvesting of mobilized normal cells from the peripheral circulation. The mechanism involved in dislodging cells from the marrow and forcing them into circulation is incompletely understood. HSC are normally anchored within the marrow environment, presumably through specific receptor interactions with other cells and extracellular matrix. In agreement with that notion, Plerixafor, an inhibitor of the stem cell homing receptor CXCR 4, has been demonstrated to improve mobilization and has recently received approval in the United States [73]. Ongoing studies are investigating optimization of patient selection for the addition of plerixafor during mobilization.
PBSC (and cells at various stages of differentiation) are harvested from peripheral blood by leukapheresis. The best currently available marker of the cells required for a successful transplant (stem cells; long-term repopulating cells) is the surface glycoprotein, CD34. For autologous procedures the goal is to harvest approximately 3–5 × 106 CD34+ cells/kg recipient weight [74]. Higher cell numbers are targeted if the cells are to be processed in vitro or if cells for a “back-up” infusion or second transplant are desired. The yield of CD34+ cells per apheresis (usually 10–16 l over 2–4 h) for autologous transplants varies considerably, largely dependent on the intensity of prior therapy given to the patients. The most reliable indicator of the success of apheresis is the concentration of CD34+ cells in peripheral blood [75]. In many patients, one single harvest will suffice; in others, several sessions are required. However, after 2 (or 3) days of apheresis, generally the incremental cell yield of CD34+ cells from additional aphereses is small. Large-volume apheresis may be useful in some patients. Usually autologous cells need to be cryopreserved until completion of the patient’s conditioning regimen.
Mobilization of PBSC by G-CSF in healthy allogeneic donors results in more consistent cell yields than in pretreated patients. By and large, 2–20 × 106 CD34+ cells/kg are obtained with a single harvest [55, 56]. As the harvest can be timed such that it coincides with completion of the transplant conditioning regimen in the patient, allogeneic PBSC are usually transplanted fresh. Cryopreservation is possible, however, and has been used, for example, to accommodate donor preferences.
Cord Blood Cells
Cord blood is collected either by venipuncture in the third stage of labor while the placenta remains in utero or by gravity drainage of the ligated umbilical vein once the placenta has been delivered [76]. Collected cord blood must be shipped to a bank for further processing and cryopreservation. At the bank, units are screened for size, and appropriate units are processed. Depending on individual bank criteria, minimum size thresholds for further processing are typically approximately 0.9 × 109 total nucleated cells (TNC) (the minimum size unit currently funded by the Health Resources and Services Administration (HRSA) in the United States). Processing strategies include directly freezing the unit in 10% DMSO vs. various approaches involving red blood cell and plasma depletion. Units may be manually depleted using hydroxy ethyl starch or PrepaCyte-CB or by automated systems including Sepax (Biosafe, Eysins, Switzerland) and AutoExpress (AXP) (Thermogenesis). Though direct freezing results in the lowest loss of total nucleated cells, the large product volumes occupy significant storage space, can cause hemodynamic complications upon infusion in small patients, and result in potentially larger DMSO exposure of patients. RBC and plasma-depleted products reduce these concerns, but some cell loss is inevitable during processing [77].
A variety of strategies is utilized by transplant centers to prepare cryopreserved units for infusion. The thaw and wash technique developed by Rubinstein et al. is most common [78]. More recently, a thaw and dilute procedure has been advocated as a strategy to increase efficiency and potentially improve infused cell doses [79]. Some centers directly thaw and infuse cord blood at the bedside.
As the number of UCB transplants has increased, banking of cord blood has increased as well. There are currently more than 100 active public cord blood banks worldwide with an inventory of greater than 400,000 units [80]. In order to donate to a public cord blood bank, an individual must deliver at a hospital that is affiliated with a public bank. A significant private cord blood banking industry has also developed. For a collection and storage fee, parents may bank their child’s cord blood for future personal use. However, given current indications for cord blood transplantation, uses of privately banked cord blood are extremely limited. Additionally, private banks are not currently regulated, and the quality of units collected is not guaranteed. Though the future potential uses of privately banked cord blood, particularly for regenerative medicine, cannot be predicted, expert opinion does not currently encourage private cord blood banking [81].
Fetal Liver Cells
Fetal liver, if obtained at the optimum time of gestation, provides a rich source of HSC [82]. The concentration of CFU-GM, an indicator for hematopoietic potential, increases with gestational age, most markedly after week 10. However, beginning at about week 14 there is also an increase in cells expressing T-cell markers. Thus, an optimum window for the use of fetal liver cells exists at 10–14 weeks of gestation. The feasibility of fetal liver transplants has been shown in adult primate and canine models as well as in in utero transplants in sheep [21, 83]. While fetal liver cells have been used successfully as intrauterine (fetal) therapy for children with immune defects [82, 84], for reasons of availability, limited numbers, technical problems, and ethical concerns, fetal liver cells have fallen out of favor and are no longer being used clinically.
Clinical Data
Since the first HSCT of the modern era was performed in 1957, the use of HSCT has increased exponentially. Starting with allogeneic (and syngeneic) marrow from related donors, the donor pool has been expanded to include unrelated volunteers, PBSC, cord blood, as well as the patient’s own marrow. Currently 25,000–30,000 allogeneic and an even larger number of autologous transplants are carried out worldwide annually with a progressively increasing proportion of transplants using PBSC and unrelated donors.
Autologous Transplantation
Malignancies
The role of autologous transplantation in the treatment of malignancies is evolving. Autologous transplantation is well established as potentially curative in relapsed/refractory aggressive NHL and germ cell tumors, and its role as upfront therapy in high-risk aggressive NHL is under investigation [85, 86]. For multiple myeloma, autologous transplantation is currently recommended for appropriately fit patients based on evidence that it increases complete remission rates and prolongs time to progression, but it is not considered curative [87, 88]. Investigation of the possible efficacy of tandem autologous transplants in multiple myeloma is ongoing [89]. The use of autologous transplantation to treat indolent lymphomas and acute leukemias is more controversial [90]. For indolent lymphomas, autologous transplantation may be used in relapsed/refractory cases, but it is generally not considered curative [91]. For acute leukemia, the lack of GVT leads to high rates of relapse, and autologous transplantation is generally not considered to improve outcomes vs. intensive chemotherapy alone [92].
Future strategies to improve autologous transplantation for malignancies include further optimizing disease-specific conditioning regimens and potentially improving methods to purge grafts of residual malignant cells—though to date extensive investigation of purging has not yielded significant improvements in outcomes [93]. Autologous transplantation is also being investigated in conjunction with planned low-intensity conditioning for allogeneic transplantation in relapsed/refractory NHL and multiple myeloma [94, 95]. The rationale is to separate the toxicity of high-intensity conditioning and allogeneic transplantation, and use a safer autologous transplant following intensive “debulking” to minimize disease burden. The minimal disease state will then hopefully allow sufficient time for a GVT effect to develop and to be sufficiently potent to render cure following low-intensity conditioning allogeneic transplantation.
Autoimmune Disorders
Several case reports and small series studies in the late 1990s suggested efficacy of autologous transplantation for scleroderma, multiple sclerosis, rheumatoid arthritis, juvenile idiopathic arthritis, systemic lupus erythematosus, and Crohn’s disease. A 2005 analysis from the EBMT summarizing results in 473 patients undergoing transplantation between 1995 and 2003 at 110 centers demonstrated 89% survival with a median follow-up of 20 months and 7% transplant-related mortality. Among 299 evaluable patients, 81% showed treatment responses with 71% sustained responses [96]. Currently a phase III randomized trial is ongoing in the United States to examine the efficacy of autologous transplantation vs. medical management for patients with scleroderma. Trials in both the United States and Europe are enrolling or planned to further investigate the potential of autologous transplantation in autoimmune disorders.
Gene Therapy
Efforts to ex vivo transfer a therapeutic gene (via a viral vector) into autologous HSC appear now close to general clinical application. Initial efforts to treat primary immune deficiencies with transfected gene-corrected HSC did not result in long-term engraftment of sufficient gene-corrected progenitors to have clinical efficacy [97, 98]. The addition of RIC (typically using busulfan) prior to infusion of the gene-corrected cells presumably opened marrow space and allowed multilineage reconstitution with sufficient corrected cells to convey clinical benefit [99]. A major concern with gene therapy, however, has been insertional mutagenesis leading to clonal expansion of transplanted cells. Early trials of gene therapy, to treat X-linked severe combined immunodeficiency (SCID-X1), resulted in clinical efficacy, but 5 of 20 patients developed T-cell clonal proliferation 2–6 years after treatment [100–102]. One patient died of refractory acute leukemia. Similarly, in the first gene therapy study to treat chronic granulomatous disease utilizing RIC prior to infusion, both patients treated developed clonal expansion of transduced cells with subsequent monosomy 7 myelodysplasia [103]. Efforts to improve the safety of gene therapy have focused on developing vectors with a reduced risk of insertional mutagenesis. Most recently, several trials have reported successful treatment of patients with adenosine deaminase (ADA)-deficient SCID without evidence of clonal proliferation [104, 105].
Allogeneic Transplantation
General complications associated with allogeneic transplantation include graft failure, delayed engraftment, regimen-related toxicity, GVHD, infection, and relapse. Clinical data regarding each of these issues as well as general outcomes with respect to donor source are discussed later and summarized in Table 57.5.
Table 57.5
Complications of transplantation by transplant type
Donor source/conditioning regimen | Graft failure | GVHD | Infection | Relapse |
---|---|---|---|---|
Autologous | b | N/A | b | b |
Allogeneic | ↑ | ↑ | ↓ | |
MRD | b | b | b | b |
MURD | b | ↑ | ↑ | ↓ |
MMURD | ↑ | ↑↑ | ↑↑ | ↓↓ |
Cord | ↑↑ | b | ↑↑ | ? ↓↓↓a |
Haploidentical | ↑↑ | ↑ | ↑↑ | ↑ |
High-intensity | b | b | b | b |
Reduced-intensity | ↑ | Similar (later onset) | ? ↓ | ↑ |
Graft Failure and Delayed Engraftment
Though graft failure is not a significant issue with HSCT from HLA-matched related or unrelated donors following high-intensity conditioning, it remains an issue when cord blood is the source of stem cells, with haploidentical and other HLA nonidentical transplants, and following RIC. Delayed engraftment of neutrophils and platelets is an additional significant concern with UCB transplants following high-intensity conditioning.
Early studies of UCB transplantation demonstrated a relationship between infused cell dose, HLA matching, and likelihood of engraftment [106–108]. A recent large analysis of single-unit UCB transplantation confirmed a relationship between HLA matching and cell dose, and suggested that greater mismatching requires greater cell doses to ensure reliable engraftment [109]. Based on accumulated evidence, current standards for acceptable UCB transplantation include at least four of six matching at low resolution for HLA-A and B and high resolution for HLA-DR antigens, as well as TNC of at least 2.5 × 107/kg. Few adult patients have single cord units available that meet minimum size criteria. To overcome this challenge, the University of Minnesota pioneered a strategy of combining two different cord units at the time of transplant to increase infused cell doses, and double-unit UCB transplants appear to have improved engraftment rates for adults [110]. Many centers incorporate algorithms for unit selection that consider both HLA-typing and unit size.
An additional concern with UCB transplantation following high-intensity conditioning remains delayed engraftment. Median time to engraftment with UCB transplantation is 24 days, as compared to 14 days with PBSC and 19 days with bone marrow (Fig. 57.1) [111]. A portion of patients undergoing UCB transplantation will experience significantly delayed engraftment; time to engraftment has not been significantly shortened with double cord transplants.
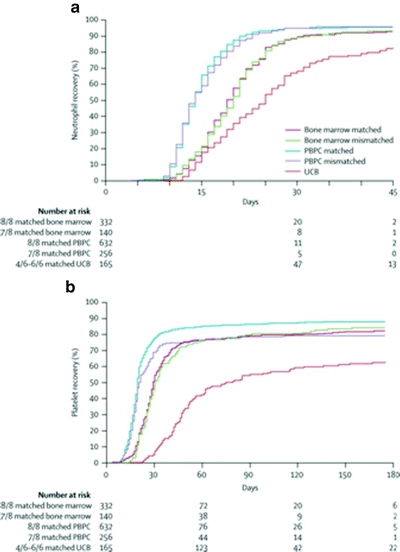
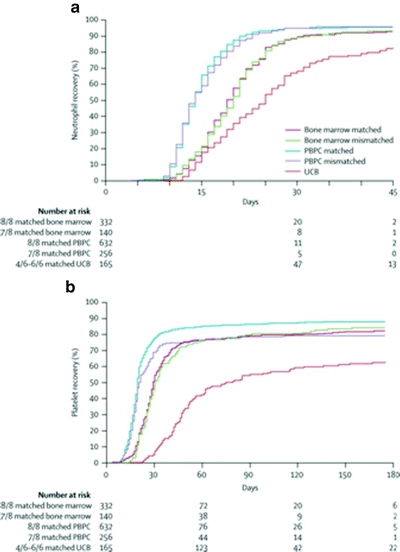
Fig. 57.1
Kinetics and probabilities of neutrophil recovery (a) and platelet recovery (b) by hematopoietic stem cell source following high-intensity allogeneic transplantation for acute leukemia. (Modified with permission from Eapen M et al. Effect of graft source on unrelated donor haemopoietic stem-cell transplantation in adults with acute leukaemia: a retrospective analysis. Lancet Oncol 2010;11(7):653–60)
Numerous additional strategies are under investigation to improve engraftment rates and decrease time to engraftment with UCB. While cell count and HLA matching are currently used to select cord blood units for transplantation, more reliable markers of the engraftment potential of individual units are desired. Assays to assess CD34+ counts and colony-forming units (CFUs) prior to cryopreservation have proven challenging to standardize across banks, and they are currently not reliable tools to predict units’ potency. Preliminary data suggest that the number of aldehyde dehydrogenase (ALDH) bright cells present in a cord blood unit may predict potency [112], and a trial investigating the reliability of a simple flow cytometric assay for ALDH bright cells will soon begin. A variety of strategies to enhance homing of cord blood stem cells is also under investigation. Preliminary evidence suggests that direct intra bone marrow injection of cord blood cells may enhance engraftment, but logistical challenges are significant. Fucosylation, inhibition of CD26, and use of prostaglandin E2 are all under investigation as strategies to improve homing and engraftment [113–117].
Other strategies involve increasing the infused number of stem cells. Fernandez et al. pioneered a strategy of co-infusing third party enriched CD34+ cells at the time of single-unit UCB transplantation [118]. The third party cells act as a bridge during the period of neutropenia, may facilitate engraftment, and provide a platform for additional manipulations to enhance immune reconstitution and GVT effects. Based on early reports of success, other centers are investigating this strategy. Efforts at ex vivo expansion of cord cells are discussed separately next.
Graft failure is also a particular concern following haploidentical transplantation. The marked mismatching sets up significant immunologic barriers, and concern about GVHD has led investigators to be reluctant to provide a large donor T-cell innoculum. Initial outcomes utilizing T-cell-depleted haploidentical donor cells resulted in high rates of graft failure. More recently, however, novel approaches to haploidentical transplantation have resulted in more reliable engraftment. The Perugia team has developed a strategy of high-intensity conditioning followed by mega-doses of G-CSF-mobilized, CD34+-selected haploidentical cells (17.5 ± 5 × 106 CD34+ cells/kg) [119]. Other groups have investigated RIC regimens combined with a variety of strategies to deplete T cells in vivo [120–122]. Among the most promising strategies, the Johns Hopkins and Seattle teams have tested an approach which administers early posttransplantation cyclophosphamide aimed at eliminating nascent proliferation of activated T cells that would cause GVHD [123].
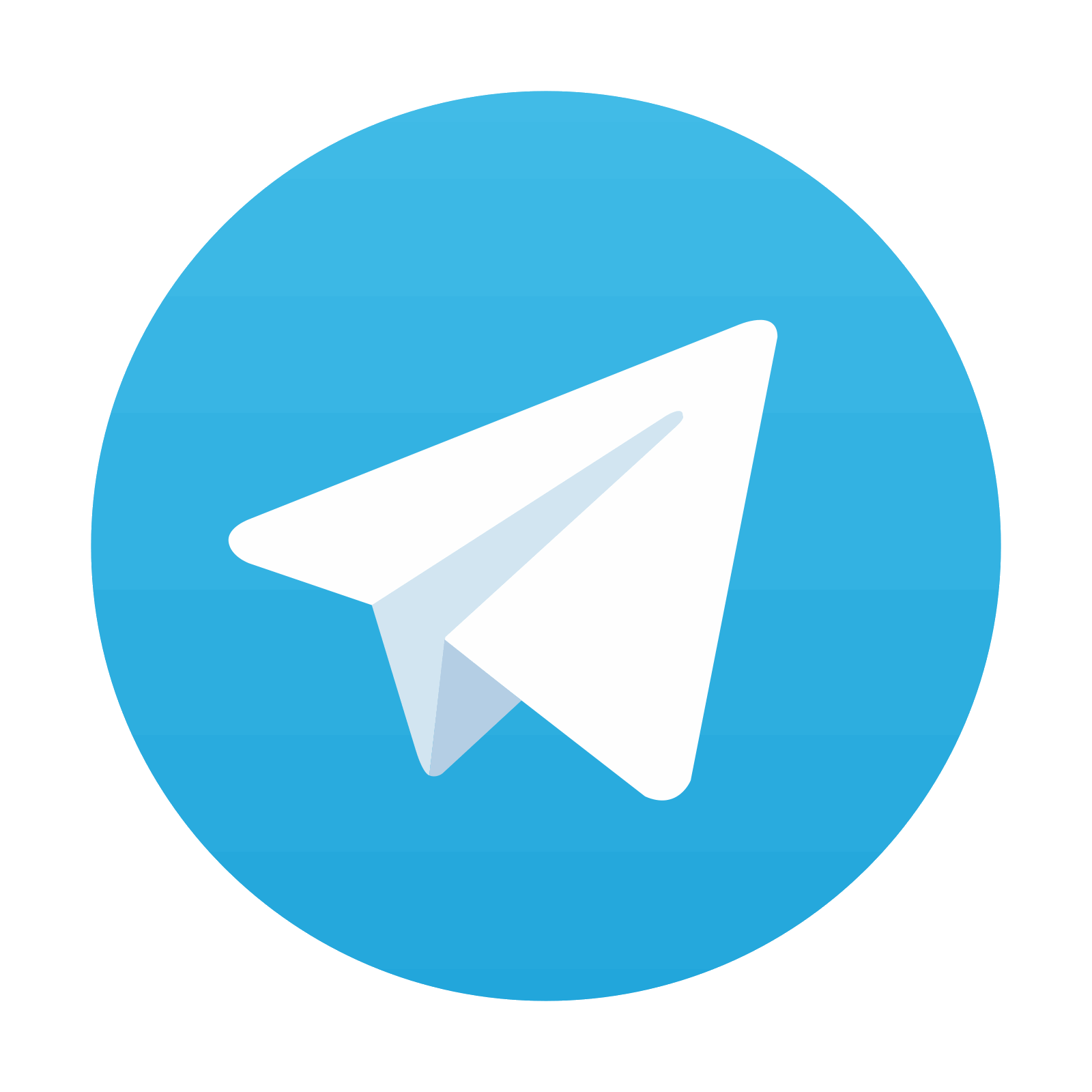
Stay updated, free articles. Join our Telegram channel
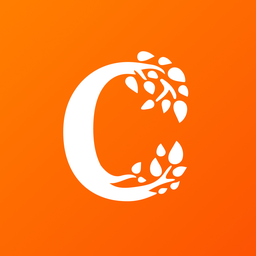
Full access? Get Clinical Tree
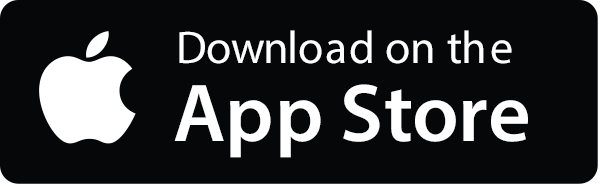
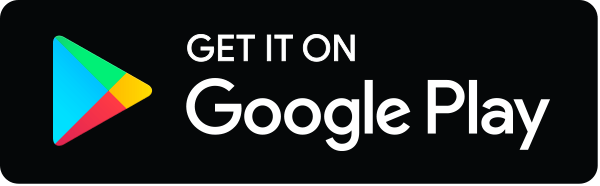