Aerospace Toxicology and Microbiology
John T. James
A. J. Parmet
Duane L. Pierson
All substances are poisons: There is none which is not a poison. The right dose differentiates a poison and a remedy.
Paracelsus (Philippus Aureolus Theophrastus Bombastus von Hohenheim, 1493-1541).
INTRODUCTION TO TOXICOLOGY
Toxicology dates to the very earliest history of humanity with various poisons and venom being recognized as a method of hunting or waging war with the earliest documentation in the Evers papyrus (circa 1500 BCE). The Greeks identified specific poisons such as hemlock, a method of state execution, and the Greek word toxos (arrow) became the root of our modern science. The first scientific approach to the understanding of poisons and toxicology was the work during the late middle ages of Paracelsus. He formulated what were then revolutionary views that a specific toxic agent or “toxicon” caused specific dose-related effects. His principles have established the basis of modern pharmacology and toxicology. In 1700, Bernardo Ramazzini published the book De Morbis Artificum Diatriba (The Diseases of Workers) describing specific illnesses associated with certain labor, particularly metal workers exposed to mercury, lead, arsenic, and rock dust. Modern toxicology dates from development of the modern industrial chemical processes, the earliest involving an analytic method for arsenic by Marsh in 1836. Industrial organic chemicals were synthesized in the late 1800s along with anesthetics and disinfectants (1). In 1908, Hamilton began the long study of occupational toxicology issues, and by World War I the scientific use of toxicants saw Haber creating war gases and defining time-dosage relationships that are used even now (2).
Aviation
The advent of aviation in World War I also saw the first use of toxins affecting pilots and ground crew. In particular, the use of castor oil as a lubricant required pilots in open cockpit aircraft to wear goggles for eye protection, and scarves around their mouths to reduce ingestion and inhalation, but the nauseating effects of this compound could not be avoided (3). Early ground support also involved hazardous chemicals causing “airplane dope poisoning” due to butyl acetate, ethyl acetate, and isopropyl alcohol inhalation (4). The modern industrial complex that supports aviation requires the use of complex and often dangerous chemicals and industrial processes, which can adversely affect the health of workers in the production, maintenance, and basic operations of an aircraft. The industrial base employs many times more people than are occupied as aircrew. Aircrew themselves may be exposed to toxicants in the course of routine operations, but are at greater risk during mishaps. The need to supply a portable form of energy to propel aircraft and spacecraft necessitates the use of high-energy fuels and hazardous oxidizers, which in the course of handling and use creates certain unique toxic hazards to the humans and the environment (5).
Space Flight
The conduct of space flight in sealed capsules and the use of reactive compounds for propulsion have caused toxicologic concerns from the earliest days of human space flight (6). Before the first Apollo moon landing, the National Academy of Sciences (NAS) released a long report recommending that submarine air quality standards for 90 days of continuous exposure be adopted for spacecraft, and that proposed limits for 60 minutes and 1,000 days of exposure be considered provisional for selected compounds (7). In 1972, the National Research Council (NRC) published limits on 52 compounds for exposure durations from 60 minutes to 6 months, with a few limits set for 10 minutes of exposure (8). The National Aeronautics and Space Administration (NASA) continued to set and revise its spacecraft maximum allowable concentrations (SMACs) independently of any outside expert
panel; however, by 1989 it was apparent that the NRC should be engaged again to formally review and set air quality guidelines for the planned space station. Since then, NASA and the NRC have maintained a partnership to set and fully document exposure guidelines for air and water quality. Most recently, these limits are being extended once again to 1,000 days as planning begins for long-duration missions to the moon and Mars.
panel; however, by 1989 it was apparent that the NRC should be engaged again to formally review and set air quality guidelines for the planned space station. Since then, NASA and the NRC have maintained a partnership to set and fully document exposure guidelines for air and water quality. Most recently, these limits are being extended once again to 1,000 days as planning begins for long-duration missions to the moon and Mars.
The Apollo 1 (originally numbered 204) fire in January 1967 on the pad at the Kennedy Space Center resulted in the death of three crewmembers in part due to toxic exposures to combustion products that were thought to include carbon monoxide, carbon dioxide, and irritant gases (9). A few years later, an Apollo crew was exposed to nitrogen tetroxide fumes as the capsule descended through the Earth’s atmosphere after a successful low Earth orbit rendezvous with a Soyuz capsule in 1975 (10). Other dramatic events in the toxicologic history of space flight include the refrigerator motor failure aboard STS-40 in which copious amounts of formaldehyde were generated from pyrolyzed Delrin polymer (11); the solid fuel oxygen generator (SFOG) fire aboard Mir in 1997 that produced little carbon monoxide but did generate organic compounds including benzene; the pyrolysis event 1 year later in the microimpurity filter that produced hundreds of parts per million (ppm) of carbon monoxide and elicited delayed headache and nausea in the crew; the persistent leaks of ethylene glycol from the thermal loops of the Mir; and the ill-fated regeneration of the metal oxide canisters used during extravehicular activity aboard the International Space Station (ISS) that caused the crew to take refuge in the Russian segment of the ISS for 30 hours to avoid the noxious vapors from the canisters regenerated in the U.S. segment (12).
Toxicologic risks associated with space flight have been a challenge to manage because of the diversity of sources, the risks from pyrolysis of polymeric material, and the limited options available to deal with such events. Despite a concerted effort toward space flight safety, it is reasonable to anticipate that such events will continue to occur as we endeavor to fly a complex ISS with an expanded number of crew, fly sorties and long-duration flights to the moon, and begin Mars exploration by humans.
BASIC PRINCIPLES OF TOXICOLOGY
Potency
A poison may be defined as an agent capable of producing an adverse effect. Virtually every chemical, including water, can produce an adverse effect in sufficient amounts. Toxic agents can be classified by the potency or relative dose required to elicit a specific adverse effect. This creates a spectrum of poisons with potencies differing by many orders of magnitude. A relative degree of toxicity can be expressed by comparing lethal doses (LDs) in six categories. For example, supertoxic agents require a dose of less than 5 mg/kg of body mass to produce death in half of those exposed, a concept expressed as the LD50. Examples of supertoxic chemicals are botulism toxin and nicotine. An extremely toxic poison requires between 5 and 10 mg/kg for an LD50, for example, the organophosphate pesticide malathion. A very toxic chemical has an LD50 from 50 to 500 mg/kg, with an example being phenobarbital. A moderately toxic agent has an LD50 in the 500 to 5,000 mg/kg range. An example would be table salt, sodium chloride. A slightly toxic chemical with an LD50 of 5,000 to 15,000 mg/kg is ethyl alcohol. A nontoxic agent, such as water, requires more than 15,000 mg/kg to produce an LD50 (13).
Route of Exposure and Target Organs
Except for contact toxicants, the initial step in exposure is absorption, which is the process by which a toxicant enters the body. Absorption can occur through various pathways. Inhalation is the most common pathway seen in the aerospace environment with vapors (gaseous component), fumes (oxides of metals), and solid particles entering the respiratory system. Water solubility determines the depth of penetration into the respiratory system. The highly soluble gas, sulphur dioxide (SO2), is readily absorbed in the nose, whereas the poorly soluble gas, nitrogen dioxide (NO2), penetrates deeply into the lung where serious injury can ensue. For fumes and dust particles, aerodynamic size determines the depth of penetration. Under conditions of nasal breathing, particles larger than 5 μm are typically captured in the nasopharyngeal region, those in the size range of 1 to 5 μm in the tracheobronchial region, and those less than 1 μm in the alveolar region. These particles may be removed from the lungs by the mucociliary escalator, by dissolution, or by the lymphatic drainage system. Smaller particles may penetrate to the alveoli and will be absorbed through epithelial membranes into the body (14). Special forms of particles, such as fibers (asbestos, carbon fibers), are measured by their cross section so that a long, hair-like particle traveling lengthwise acts like a small particle based on its cross sectional area and not on its length.
Chemicals enter the body through routes other than the pulmonary system. The eyes and nasal mucosa are nonkeratinized epithelium and readily absorb water-soluble particles and respond to acids and bases. The skin, however, is waterproof and lipid proof, and highly resistant to absorption of most chemicals. Only chemicals with unique polarity, such as dimethyl sulfoxide, can penetrate intact skin, although burns and infections may break down the keratinized layer and permit significantly increased amounts of chemicals to cross the dermal barrier and enter the body. Ingestion through the gastrointestinal tract provides opportunities for chemicals requiring acidic environments (the stomach) or alkaline environments (esophagus and duodenum) to be solubilized and absorbed. Finally, some chemicals may be injected intentionally across the skin in a parenteral manner through accidents or medical procedures.
The next phase of exposure is called distribution. Chemicals will be dispersed throughout the body, often redistributing on the basis of their pH-based solubility or
solubility in fat. Those chemicals that tend to dissociate in an acid environment such as aspirin will concentrate in low pH areas such as the stomach or joint spaces even when absorbed by another mechanism. Therefore, aspirin taken as an enteric-coated capsule and absorbed in the small intestine will eventually distribute back into the stomach. Other chemicals will distribute by the nature of their binding capacity for blood proteins, especially albumin. Proteinbound toxicants are not available to disperse into other tissues until they have been freed by dissociation. Metals such as lead will be carried on specific proteins designed as carriers for normal metabolic components. In the case of lead, erythrocyte protoporphyrin, normally used to transport zinc, will carry the chemically similar lead where it will bind in place of zinc and calcium.
solubility in fat. Those chemicals that tend to dissociate in an acid environment such as aspirin will concentrate in low pH areas such as the stomach or joint spaces even when absorbed by another mechanism. Therefore, aspirin taken as an enteric-coated capsule and absorbed in the small intestine will eventually distribute back into the stomach. Other chemicals will distribute by the nature of their binding capacity for blood proteins, especially albumin. Proteinbound toxicants are not available to disperse into other tissues until they have been freed by dissociation. Metals such as lead will be carried on specific proteins designed as carriers for normal metabolic components. In the case of lead, erythrocyte protoporphyrin, normally used to transport zinc, will carry the chemically similar lead where it will bind in place of zinc and calcium.
Once in the body, chemicals are distributed and will contact the target organ. Highly perfused organs (e.g., liver) will receive more of a toxicant than those less perfused. Special transport proteins concentrate selected toxicants into certain organs; for example, iodine is concentrated into the thyroid by a protein in thyroid cell membranes. Most toxicants have a specific binding site where the effect takes place. For lead, it may substitute for zinc in intracellular processes affecting the synthesis of heme or it may substitute for calcium in bone. Other chemicals, particularly strong acids or alkalies such as hydrazine, are nonspecific, and their effects are to denature proteins of all kinds causing a very nonspecific response at the binding site.
Metabolism will also affect the outcome of chemical exposure. Enzymes in the nasal mucosa and stomach will often metabolize chemicals before they enter the body. Gastric dehydrogenases break down ethyl alcohol within the stomach, and differing amounts of the enzyme such as larger amounts present in males than females, will affect the relative toxicity. Chemicals absorbed through the gastrointestinal tract undergo first-pass metabolism in the liver through the portal system. Therefore, chemicals absorbed from the stomach and intestine will travel to the liver before entering the general distribution. The importance of metabolism is illustrated in this example: cocaine ingested in the form of tea, commonly done in South America, is metabolized to benzylecgonine, a nonpsychoactive agent, and therefore very little of cocaine taken as an ingested liquid enters the system and causes psychogenic effects. When cocaine enters the body through lungs or nasal mucosa, this first-pass metabolism is not present and no transformation of the chemical and psychotic effects are observed (15). Biotransformation may also adversely change a chemical, as demonstrated by aflatoxin, which is transformed by cytochrome P-450 in the liver into a carcinogen through its first-pass metabolism (16).
Ultimately, a toxicant will be eliminated through any one of a number of excretion systems. Many chemicals are excreted through the kidneys into urine. Others may be excreted through the bile, although these toxicants may be subject to enterohepatic recirculation. An example is mercury, which is excreted in the bile by methylation in the liver. However, in the colon, intestinal bacteria will break down the methyl mercury and allow reabsorption and return to the system (17). This can prolong the effective half-life of the chemical. Other chemicals such as solvents can be excreted as vapor through the lungs, an example being ethyl alcohol. Ethanol itself, being a carbohydrate, is also metabolized to acetaldehyde, acetic acid, and carbon dioxide. Other chemicals may be excreted as deposits in the hair, skin, and nails. Examples are arsenic and cannabinoids. Some chemicals may be stored for long periods of time, particularly those soluble in fats or in bone. All these reservoirs can serve to affect the half-life of a chemical in the body and can possibly lead to toxicity if the reservoir is suddenly “emptied.” For example, as bone is mobilized during space flight, the release of other compounds stored in bone, such as lead, could cause toxicity if the amount stored in bone were sufficiently high before flight (18).
Extrapolations in Exposure Times and Species
Judgments must be made concerning the dose that a human can take without succumbing to toxicologic effects. Those judgments are formed a priori and given as exposure standards for specific times of exposure to avoid specific adverse effects. The data to set such standards is often in a nonhuman species and has not been generated for the times of exposure for which a standard is needed. To set the human exposure standard, a paradigm for extrapolation from one species to another is needed and another paradigm is needed to extrapolate from one time of exposure to another. There is by no means a consensus on how each of these extrapolations should be done. Some of the options are discussed in the subsequent text.
The most accurate method for extrapolation of animal data to the human condition is through physiologically based pharmacokinetic (PBPK) modeling. By this approach, the concentration of the ultimate toxicant (the one causing the injury) is modeled and measured where possible to estimate the differences between the tested species and the human. Physiological and metabolic parameters must be derived or assumed for each species in this approach, and the modeling must account for changing routes of metabolism as the exposure concentration increases and metabolic pathways are overwhelmed. For example, a PBPK model has been used to compare the relative blood concentrations of n-butanol (a common spacecraft pollutant) in rats and make a prediction in humans if they were exposed to a precursor of the alcohol (n-butyl acetate) (19). The target endpoint to be avoided is central nervous system depression, which can be observed in the exposed rats as reduced voluntary activity.
When extrapolating from one species to another, the toxicologist must ask whether the toxic effect observed in the animal species is relevant to human exposures. Examples where rodents are an inappropriate model for humans include the calcium mobilization response of guinea
pigs to elevated carbon dioxide, the neoplastic response of rats to thyroid carcinogens, and the hyaline-droplet accumulation in the male rat kidney during solvent exposures (20). Another example of an inappropriate endpoint is the neoplastic response of rat liver to ingestion of di (2-ethylhexyl) phthalate in water. Rodents absorb much less of the toxicant than humans after ingestion, but the peroxisome proliferative response in their livers, which is mediated by peroxisome proliferator-activated receptor-α, is nearly absent in primates (21). The toxicologist must consistently question the relevance of the modeled response to a toxicant and the expected human response.
pigs to elevated carbon dioxide, the neoplastic response of rats to thyroid carcinogens, and the hyaline-droplet accumulation in the male rat kidney during solvent exposures (20). Another example of an inappropriate endpoint is the neoplastic response of rat liver to ingestion of di (2-ethylhexyl) phthalate in water. Rodents absorb much less of the toxicant than humans after ingestion, but the peroxisome proliferative response in their livers, which is mediated by peroxisome proliferator-activated receptor-α, is nearly absent in primates (21). The toxicologist must consistently question the relevance of the modeled response to a toxicant and the expected human response.
Low-dose Extrapolation
One of the classic problems in toxicology is extrapolation of the toxic responses of an animal model at high doses to the anticipated response at much lower doses, well below any of those used in the animal study. The typical approach to this problem is to derive a dose-response curve that expresses the severity or incidence of a response to toxicant exposures. In the last decade, the benchmark dose approach has been promulgated by the NRC and others as a means of estimating low-dose responses from studies performed at higher doses (22). As the Johnson Space Center (JSC) Toxicology Group has attempted to apply this method to setting of exposure guidelines in cooperation with the NRC, three issues with its application have emerged. The first problem is the random variability in the data when the number of test animals per dose is in the vicinity of 10, which is a typical number for many studies. The second problem is how to select the model or models to fit the data, and then how to combine their predictions into a single parameter. The third problem is which statistical endpoint to use as the predictor. Should it be the lower confidence limit or the maximum likelihood value, and should the predicted risk level be 1%, 5%, or 10%? There is not a consensus on how benchmark dosing should be applied; however, it is deemed to be an improvement on the default approach of using a no-observed-adverse-effect level as a starting place for low-dose predictions.
Combined Exposures
In any actual situation, people are exposed to mixtures of compounds in the air and water. Often the toxic risk is primarily due to a single pollutant; however, at other times the combined effects of several compounds must be considered. For example, the initial step to addressing the problem of multiple-compound exposures is to calculate a toxicity index (T value) for each space mission. The calculation is as follows:
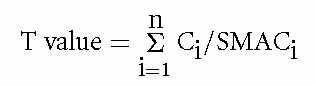
Where there are “n” compounds found at concentrations Ci and the exposure standard for the ith compound is the SMACi, which is the limit for the time the crew has been exposed. For example, for short Shuttle missions the 7-day SMACs are typically used, whereas for a prolonged stay aboard the ISS, the 180-day SMACs are used. The air is considered acceptable if T is less than 1; however, this is often not the case. When T greater than 1 is found, the compounds are sorted into groups according to their toxic mechanism or target organ. For example, irritants, carcinogens, hematotoxicants, immunotoxicants, neurotoxicants, cardiotoxicants, and so on constitute separate groups. Then a Tgroup is calculated for each group of toxicants. The air is considered safe if each Tgroup is less than 1 unit. Note that some compounds with multiple toxic effects, such as carbon monoxide, can contribute to more than one group. To apply this method, one must know a priori the target organs of each compound, and that is sometimes not well established.
Immediate versus Delayed Toxic Effects
It is important that flight surgeons and biomedical engineers supporting a flight be aware that certain toxicants do not elicit their maximum effects immediately, or the nature of the effect may change with time. A good example of this is the delayed pulmonary edema caused by nitrogen tetroxide exposures in the Apollo capsule as the oxidizer was aspirated into the capsule from thrusters. The estimated time of exposure was 4 minutes and 40 seconds at an average concentration of 250 ppm, with a peak at 700 ppm. One crewmember was unconscious when the capsule was opened. The crew experienced immediate symptoms of respiratory irritation, but the pulmonary edema (infiltrate) was delayed for about a day (10). The symptoms included chest tightness, a retrosternal burning sensation, and a cough upon deep breathing. Chest x-rays taken the following day were suggestive of chemical pneumonitis; however, these findings returned to normal 5 days after landing. The crew was treated with oral steroids. To our knowledge, no long-term health consequences have been reported in the three crewmembers.
Another example of delayed toxic effects during space flight was the carbon monoxide exposure after the microimpurity filter pyrolysis aboard Mir. When crewmembers were exposed to several hundred ppm of carbon monoxide, several hours were required for the carboxyhemoglobin to accumulate in the blood to its maximum level. Therefore, it was not until approximately 8 hours after their initial exposure that any crewmembers reported headache and nausea after the filter burn.
Adaptive Responses versus Adverse Effects
When assessing the health significance of a toxic exposure, one must clearly delineate adaptive responses of an organism from adverse effects caused by a toxicant. One example that directly applies to space flight is the anthropogenic compound carbon dioxide (CO2). The normal outdoor concentration of carbon dioxide is approximately 0.05%; therefore, it is relatively easy for people to discharge this metabolic product from the body. Hyperventilation (an adaptive response) can be demonstrated at exposure
concentrations of 1% or more, but the effect goes unnoticed by the person exposed. The increased respiratory rate is mediated by chemoreceptors in the carotid and brain; however, if the exposure is prolonged, it appears that the hyperventilation fades as the person acclimates to the high CO2 during weeks of exposure (see Chapter 2). Substantially higher concentrations (>3%) are generally required to elicit clearly adverse effects such as headaches, dyspnea, or intercostal pain (23). Spacecraft are operated to maintain the concentration below 0.7% (7,000 ppm), but there may be sensitive crewmembers who are susceptible to headaches even at this low concentration.
concentrations of 1% or more, but the effect goes unnoticed by the person exposed. The increased respiratory rate is mediated by chemoreceptors in the carotid and brain; however, if the exposure is prolonged, it appears that the hyperventilation fades as the person acclimates to the high CO2 during weeks of exposure (see Chapter 2). Substantially higher concentrations (>3%) are generally required to elicit clearly adverse effects such as headaches, dyspnea, or intercostal pain (23). Spacecraft are operated to maintain the concentration below 0.7% (7,000 ppm), but there may be sensitive crewmembers who are susceptible to headaches even at this low concentration.
Individual Sensitivities (Genetic Factors)
Individuals can vary in their response to toxic insult because of age, health status, previous exposure, or genetic differences. People who are very young or very old are generally considered to be more susceptible to environmental toxicants. Persons with respiratory disease are likewise more susceptible to air pollutants and are often warned to remain inside when air quality in urban areas is poor. Previous exposures can either increase a person’s sensitivity to subsequent exposure or decrease it. In occupational asthma and reactive airways disease, once an allergen has sensitized an individual, subsequent reexposure will evoke a symptomatic response at a much lower level (24). On the other hand, smokers, human test subjects, and even experimental animals that experience multiple exposures to carbon monoxide have adapted to it, and are therefore less susceptible to some of the adverse effects of carbon monoxide than naîve organisms (25).
Our understanding of the genetic basis for differences in susceptibility of individuals to toxic insults is being revolutionized by the new field of toxicogenomics, which is the study of all genes of a cell or tissue at the DNA, messenger ribonucleic acid (mRNA), or protein level (26). Before the era of toxicogenomics, there had been long-standing recognition that certain persons were much more susceptible to adverse effects when exposed to certain toxicants. An example that applies to aviation and to space flight is that of ethanol. Ingestion of ethanol is discouraged in both settings, yet we must recognize that certain individuals are extremely prone to the “alcohol sensitivity syndrome,” because of genetic polymorphisms in the enzymes that catalyze the removal of ethanol’s metabolite, acetaldehyde. In sensitive individuals, this compound causes an unpleasant flushing response, which includes facial redness, increased pulse rate, headache, nausea, and drowsiness, even with a single ingestion of a small amount of ethanol (27). Toxicogenomics facilitates our understanding at the molecular level of the effect of a toxicant on the genome as well as our understanding of individual variability in susceptibility because of their genetic predisposition to be adversely affected by exposure to a compound. In principle, each person could be screened for genetic markers that suggest their level of susceptibility to the adverse effects of a drug or toxicant.
SPECIFIC CHEMICALS IN THE AEROSPACE ENVIRONMENT
Aviation Fuels and Compounds
Liquid aviation fuels are primarily petroleum-based compounds. In general, all aviation propellants consist of mixtures of alkanes, cycloalkanes, and other hydrocarbons in varying ratios. All are volatile and flammable. Petroleum-based fuels fall into two categories: aviation gasoline or jet fuel. In most locations across the globe, aviation gasoline or avgas no longer contains tetraethyl lead, which was removed for environmental protection. Most jet fuel is a blend of kerosene with specific additives to produce characteristic performance. Each manufacturer of avgas and jet fuel will blend approximately 300 hydrocarbon compounds to produce the energy requirements, controlled oxidation, and inhibition of corrosion and freezing necessary for specific applications. Blends are adjusted for seasonal changes in weather and to control “weathering” of stored fuels in specific locations (5).
Fuels can cause skin irritation, and the vapors will cause nausea and sedation. Long-term inhalation can lead to neuropathies and are suspect for hepatotoxicity and carcinogenicity (28). However, most fuels are now handled by a single-point system. This means that the fuel line must be locked and sealed between the tankers and aircraft so that no fuel will flow until the system is closed. This produces very minimal exposure to fuel vapors in the occupational setting (29). Combustion products of fuels include carbon monoxide, carbon dioxide, incompletely oxidized hydrocarbons, and oxides of nitrogen generated by heating of atmospheric nitrogen. The exhaust of internal combustion engines using aviation gas tend to be rich in carbon monoxide whereas jet fuel burning gas turbine engines are typically low in carbon monoxide, but may be high in oxides of nitrogen.
Other petroleum products encountered in the aviation environment include lubricants, which are typically heavier oils and hydraulic fluids. Some hydraulic fluids have phosphate components, and under certain circumstances such as combustion highly toxic organophosphates may be generated. Despite this concern, there is no consistent proof that such events have occurred; nonetheless, such fluids have generally had the phosphate esters substituted (30). De-icing fluids may contain ethylene glycol or propylene glycol, which have the potential to be nephrotoxic and must be recovered to prevent environmental contamination (31).
Rocket Fuels
Rocket fuels may consist of kerosene (RP1) used in the Atlas missile, liquid hydrogen which is used for the main engines of the space shuttle and upper stages of other missiles, or hydrazine (32). The toxicologic properties of kerosene are similar to jet fuels. Hydrogen is a nontoxic gas that is a cryogenic liquid at −255°C. Hydrazines are used as the fuel in hypergolic engines. This fuel when mixed with an oxidizer will spontaneously combust. Hydrazines are available as
hydrazine (H2N-NH2), monomethyl hydrazine (MMH) (H2N-NHCH3), and unsymmetrical dimethyl hydrazine (UDMH) (H2N-N(CH3)2). Hydrazines are used as the fuel for the space shuttle orbiter maneuvering system and reaction control systems and as the power supply for the auxiliary power unit on F-16 fighters. In the F-16, MMH is passed through a catalytic converter, which decomposes hydrazine to ammonia and steam (33). Hydrazines are very stable, clear, and colorless liquids with an ammonia-like odor. However, hydrazine is much more toxic than ammonia. Ammonia has a 24-hour SMAC of 20 ppm and can be smelled at 5 ppm, whereas hydrazine is also smelled at 5 ppm, but its 24-hour SMAC is only 0.3 ppm (NASA/JSC 20584, March 2001). As highly alkaline chemicals, they rapidly penetrate intact skin and coagulate proteins. Extreme toxicity to the eyes and respiratory tract occur, and workers should have complete isolation in the form of respiratory and skin protection. Rapid decontamination with copious amounts of water is necessary, followed by symptomatic treatment of skin, eye, and airway injury. Seizures may occur following MMH absorbtion and are resistant to standard therapy. Pyroxidine treatment at 25 mg/kg has been suggested from experimental studies (34).
hydrazine (H2N-NH2), monomethyl hydrazine (MMH) (H2N-NHCH3), and unsymmetrical dimethyl hydrazine (UDMH) (H2N-N(CH3)2). Hydrazines are used as the fuel for the space shuttle orbiter maneuvering system and reaction control systems and as the power supply for the auxiliary power unit on F-16 fighters. In the F-16, MMH is passed through a catalytic converter, which decomposes hydrazine to ammonia and steam (33). Hydrazines are very stable, clear, and colorless liquids with an ammonia-like odor. However, hydrazine is much more toxic than ammonia. Ammonia has a 24-hour SMAC of 20 ppm and can be smelled at 5 ppm, whereas hydrazine is also smelled at 5 ppm, but its 24-hour SMAC is only 0.3 ppm (NASA/JSC 20584, March 2001). As highly alkaline chemicals, they rapidly penetrate intact skin and coagulate proteins. Extreme toxicity to the eyes and respiratory tract occur, and workers should have complete isolation in the form of respiratory and skin protection. Rapid decontamination with copious amounts of water is necessary, followed by symptomatic treatment of skin, eye, and airway injury. Seizures may occur following MMH absorbtion and are resistant to standard therapy. Pyroxidine treatment at 25 mg/kg has been suggested from experimental studies (34).
Nitrogen tetroxide and nitric acid are the oxidizer components in hypergolic engines and spontaneously ignite when mixed with hydrazines. These oxidizers are used in the space shuttle onboard maneuvering system as well as the upper stage for other rocket engines (35). They are highly acidic, forming nitric acid on contact with water or the human body, and will rapidly cause burns to the eyes, skin, and respiratory tract. Complete protection must occur if these oxidizers are present in the environment. Rapid decontamination with copious amounts of water is necessary, followed by symptomatic treatment of skin, eye, and airway injury. Inhalation injury may include pulmonary edema, which may be fatal or result in residual bronchiolitis obliterans (36)
Solid fuels contain a mixture of ammonium perchlorate, the oxidizer, and a metal, usually aluminum or magnesium, which is the fuel. Additionally, a binding agent such as a plastic will be included in the mixture to create a stable solid. Most air-to-air missiles and the solid rocket boosters for space shuttle use this composition. This mixture is extremely stable until ignited and the combustion products produced are hydrochloric acid and a metal oxide (37). The exhaust gases are irritating to eyes and the respiratory tract (38).
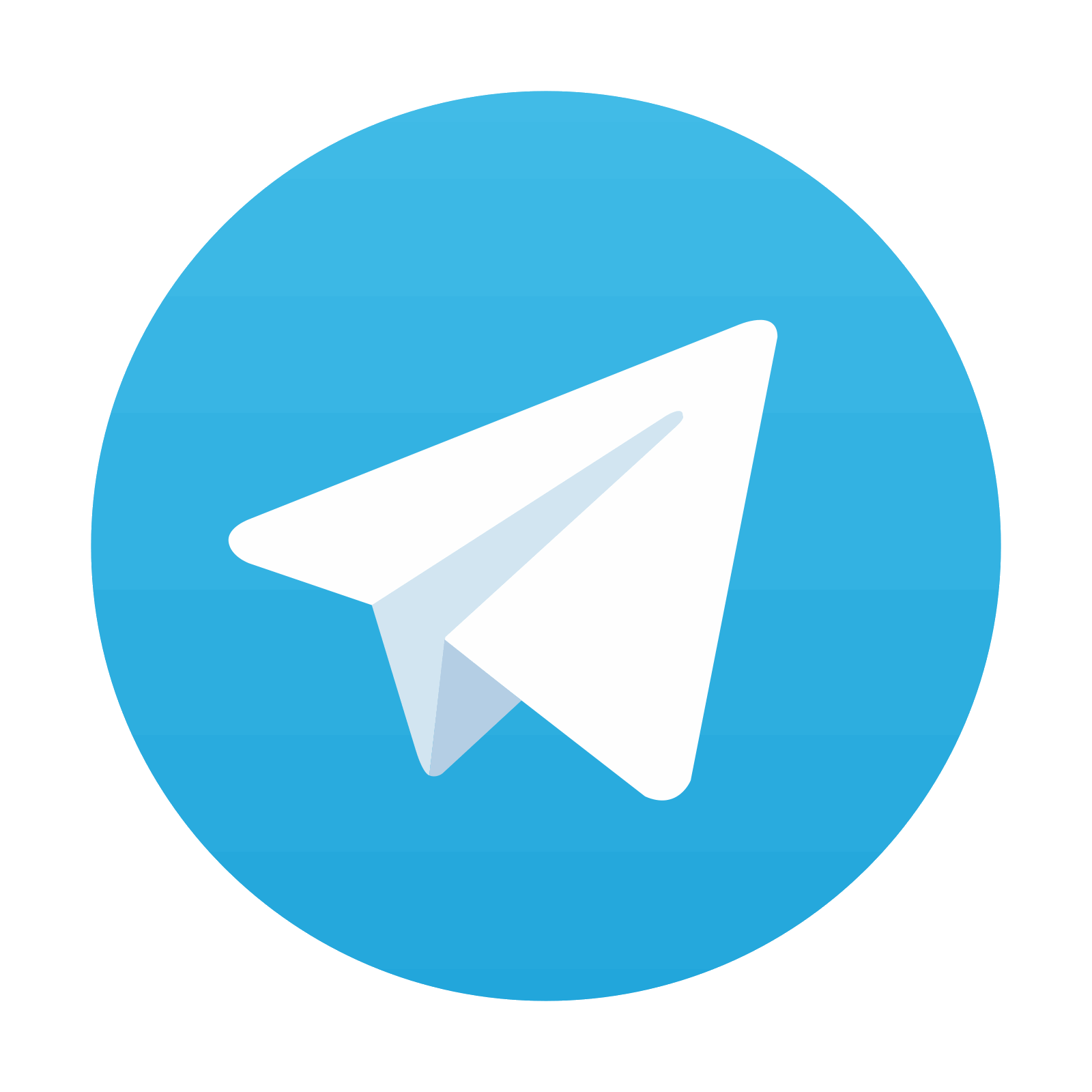
Stay updated, free articles. Join our Telegram channel
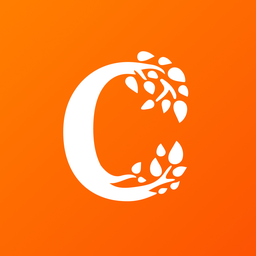
Full access? Get Clinical Tree
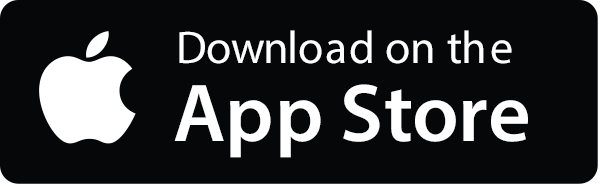
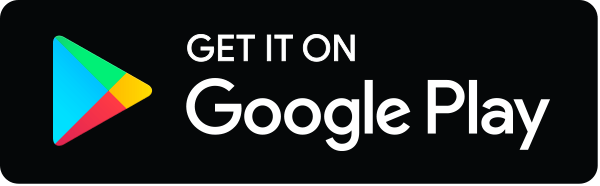