Highlights
- •
Family history of prostate cancer is an important risk factor of being diagnosed with the disease, even in the setting of low PSA.
- •
Black men are at increased risk for prostate cancer and worse outcomes.
- •
BRCA2, MSH2, MLH1, MSH6, and HOXB13 -carriers have increased prostate cancer risk.
- •
Polygenic risk scores may assist with prostate cancer risk-stratification and guide personalized screening regimens.
- •
Risk models with age, family history, and genetics offer the greatest predictive power.
Abstract
Aim
To investigate the role of family history, race/ethnicity, and genetics in prostate cancer (PCa) screening.
Methods
We conducted a systematic review of articles from January 2013 through September 2023 that focused on the association of race/ethnicity and genetic factors on PCa detection. Of 10,815 studies, we identified 43 that fulfilled our pre-determined PICO (Patient, Intervention, Comparison and Outcome) criteria.
Results
Men with ≥1 first-degree relative(s) with PCa are at increased risk of PCa, even with negative imaging and/or benign prostate biopsy. Black men have higher PCa risk, while Asian men have lower risk. Most of the differences in risks are attributable to environmental and socioeconomic factors; however, genetic differences may play a role. Among numerous pathogenic variants that increase PCa risk, BRCA2, MSH2, and HOXB13 mutations confer the highest risk of PCa. Polygenic risk score (PRS) models identify men at higher PCa risk for a given age and PSA; these models improve when considering other clinical factors and when the model population matches the study population’s ancestry.
Conclusions
Family history of PCa, race/ethnicity, pathogenic variants (particularly BRCA2, MSH2, and HOXB13 ), and PRS are associated with increased PCa risk and should be considered in shared decision-making to determine PCa screening regimens.
1
Introduction
Prostate cancer (PCa) is the most common solid organ cancer in men, with over 200,000 new cases each year in the US [ ]. While PCa is oftentimes indolent, many patients still present with advanced disease and have higher odds of morbidity and mortality. The American Urological Association (AUA) guidelines recommend PCa screening with prostate-specific antigen (PSA) with or without digital rectal exam (DRE) in men 45-69 years old [ ]. For patients outside that range, screening may be based on shared decision-making with consideration of risk factors such as Black or African American ancestry, germline mutations, and strong family history of PCa [ ]. One-size-fits-all approaches are limited, thus there have been efforts to improve screening practices and tailor screening recommendations based on individual patient risk factors.
Modifiable risk factors such as environment, diet, stress, alcohol and tobacco use have been investigated for their role in the development of PCa and are targets of PCa-prevention interventions [ ]. Non-modifiable PCa risk factors include age, family history, race/ethnicity, pathogenic gene variants, and genetic risk scores. Stratifying by these risk factors may improve identification of individuals at high risk of clinically significant PCa (csPCa). Lower-risk patients could benefit from reduced screening, over-diagnosis, unnecessary invasive procedures, and limiting healthcare costs while higher-risk patients may benefit from earlier and/or more aggressive screening and subsequently earlier csPCa detection [ ].
Here, we conducted a systematic review of studies pertaining to the role of family history, race/ethnicity, pathogenic germline mutations/variants, and polygenic risk scores (PRS) in PCa screening.
2
Methods
2.1
Literature Search Strategy
OVID was used to systematically search MEDLINE and EMBASE databases for articles evaluating risk of PCa detection in association with race or ethnicity and genetic factors. Databases were searched for studies published from January 2013 through September 2023 (Week 36). In addition to the MEDLINE and EMBASE databases search, reference lists of included systematic reviews and primary literature were scanned for potentially useful studies.
2.2
Study Selection Criteria and Process
All hits from the OVID literature search were input into reference management software (EndNote 21), where duplicate citations were removed. Abstracts were reviewed by the methodologist to determine if the study addressed the PICO (Patient, Intervention, Comparison and Outcome; Table S1 ) criteria. Randomized controlled trials, observational studies, modelling studies, and case-control studies with at least 30 patients per study arm were considered for inclusion in the evidence base. Case series, letters, editorials, in vitro studies, studies conducted in animal models, and studies not published in English language were excluded from the evidence base a priori. Full-text review was conducted on studies that passed the abstract screening phase by all authors. Studies were compared to the PICO criteria by one author and inclusion criteria was verified by the methodologist. The results are limited by numerous manuscripts providing results without distinction between clinically significant versus indolent PCa. When specified in the original manuscripts, more specific terminology was used. Low-risk PCa was defined as GG1 while csPCa or high-risk PCa was defined as GG≥2.
2.3
Data Extraction
Data were extracted from all studies that passed full-text review by the methodologist.
2.4
Quality Assessment: Individual Study Quality and Potential for Bias
Quality assessment for retained studies was conducted. Studies deemed to be low quality would not be excluded from the systematic review, but would be retained, and their methodological strengths and weaknesses discussed where relevant. To define an overall study quality rating for each included study, risk of bias as determined by validated study-type specific tools was paired with additional important quality features. To evaluate the risk of bias within the identified studies, the Assessment of Multiple Systematic Reviews (AMSTAR) [ ] tool was used for systematic reviews and a Risk of Bias in Non-Randomized Studies – of Intervention (ROBINS-I) [ ] was used for observational studies, and Prediction model Risk of Bias aSsessment Tool (PROBAST) was used for model studies [ ]. Summary risk of bias assessment figures were created using robvis [ ]. Additional important quality features, such as study design, comparison type, power of statistical analysis, and sources of funding were extracted for each study. We note that nearly all included studies have moderate or high risks of bias.
3
Results
The systematic review identified 10,815 studies. Following dual study selection comparing the studies to the predefined PICO elements, 43 studies were chosen for inclusion in the evidence base ( Figure 1 ). Studies were categorized into those that evaluated risk associated with family history, race/ethnicity, pathogenic variants, and/or polygenic risk score.
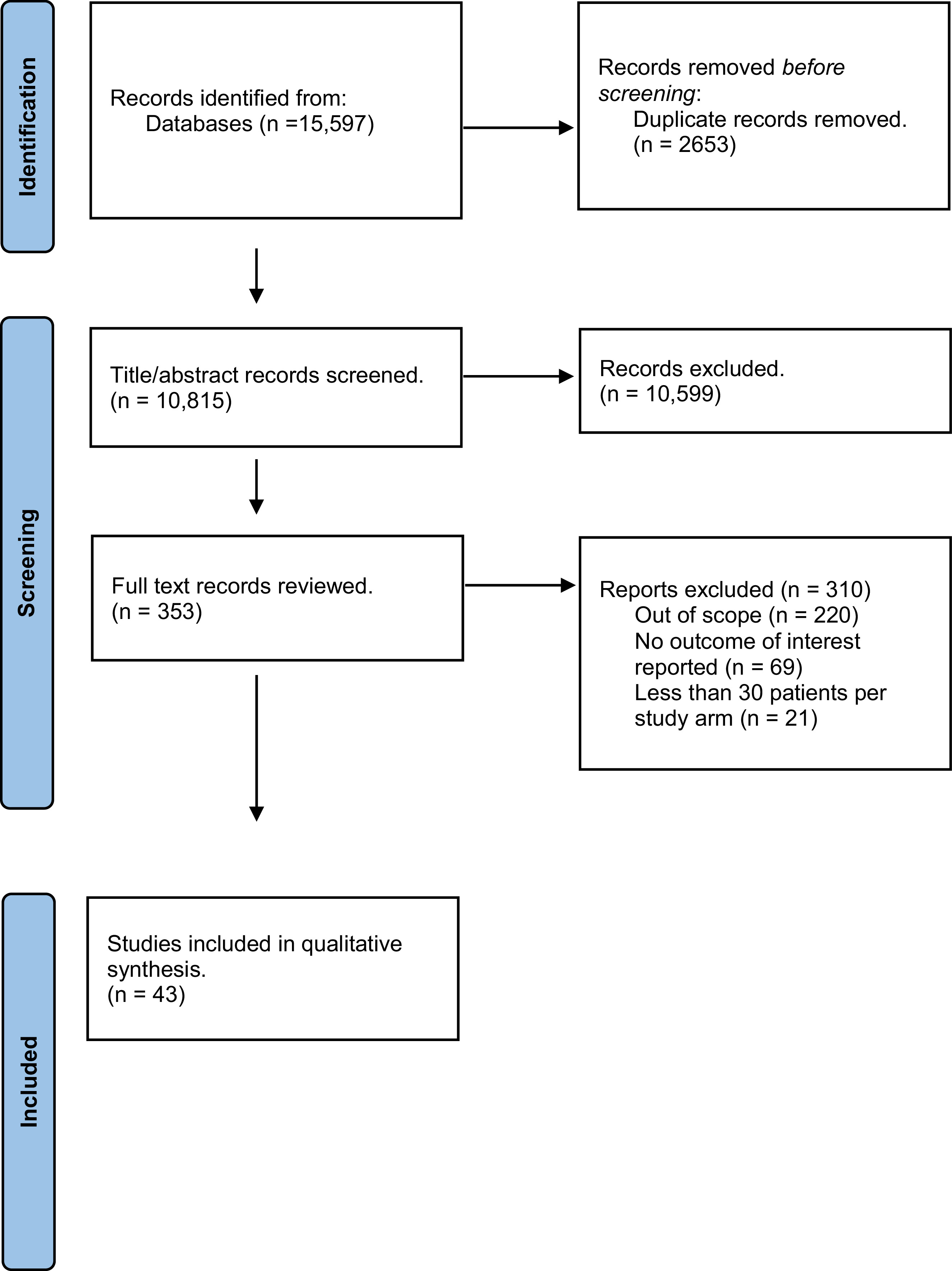
3.1
Family History
Family history (FH) of PCa is associated with being diagnosed with the disease. There were four studies included for FH [ ] ( Table S2 ). All included studies were limited by an overall high risk of bias (summarized in Figures S1-2 ). FH-positive status was based on first-degree relatives with PCa (defined as ≥GG1) in all studies, while one study [ ] also included second-degree relatives. A positive FH is associated with a 1.2-8.4-times odds of detecting csPCa on subsequent biopsy [ , ]. Prostate cancer detection was higher in FH-positive men, with risk increasing with additional first-degree relatives with the disease [ ]. Family history has also been used to improve predictive risk models [ , , ] ( Table S2 ).
3.2
Race/Ethnicity
Ten studies evaluating the association between race or ethnicity and risk of PCa detection or outcomes were included in the evidence base [ , ]. All studies carried an overall critical risk of bias based upon critical risk of bias in patient selection across all studies, plus several studies were also limited by moderate risk in other bias domains (summarized in Figure S1 ).
3.2.1
PSA plus Race/Ethnicity: Prostate Cancer Detection
Several studies reported racial disparities in PCa detection. African-American men with PCa had higher pre-biopsy PSA, higher PSA at diagnosis, higher rates of localized and de novo metastatic PCa (mPCa), and higher tumor burden compared to White men as well as higher PCa prevalence and younger age at time of diagnosis compared to other ancestries [ , ] ( Table S3 ). Black race was more strongly associated with high-grade PCa compared to White men, even after adjustment for age, PSA, DRE, and body mass index [ ]. Interestingly, Saltzman et al. (2015) found few/no racial disparities in PCa stage or outcomes amongst men aged 40-54 [ ] ( Table S3 ). Giri et al. (2022) found no differences in Gleason Scores (GS) between Black and White men [ ] ( Table S3 ). African-Caribbean ancestry was also a risk factor for csPCa in men with clinical suspicion for PCa and negative or no prior work-up [ ].
While many studies on race and ethnicity and PCa grouped patients under broad categories, some suggested dis-aggregated categories better characterize differences in genetic and environmental impacts on PCa incidence and outcomes within racial and ethnic subgroups [ ]. Jain et al. (2022) dis-aggregated Asian races and ethnicities and found some subgroups had significantly higher odds of presenting with a higher PCa risk group compared to White patients, with odds increasing by 18-400% depending on the subgroup. For example, Laotian, Hmong, and Kampuchean men were the only subgroups with greater than 50% increased odds (OR 1.60, 4.07, and 1.55, respectively) of high risk PCa. Thai, Asian Indian, and Pakistani men did not have significantly greater odds of presenting with higher risk disease [ ].
3.2.2
PSA Plus Race/Ethnicity: Prostate Cancer Mortality/Survival
Some studies focused on the association of race/ethnicity with long term PCa-related outcomes. One study showed Black men had greater prostate cancer-specific mortality (PCSM) than in non-Black men and were more likely to present with metastases, even when adjusting for demographic and clinical factors [ ]. Similarly, another study found that PSA screening in Black men was associated with a lower risk of PCSM compared to White men. Importantly, consistent annual PSA screening significantly reduced PCSM more in Black men than White men, compared to occasional screening [ ]. A multi-ethnic study of PSA levels up to 10+ years prior to PCa diagnosis revealed that average age-adjusted PSA levels were significantly different across racial/ethnic groups with Black men and Latino men having significantly higher average PSAs while Native Hawaiian and Japanese men had lower average PSAs compared to White men [ ]. Matti et al. (2021) reported lower 15-year cancer-specific survival in Māori and Pacific men compared to White men [ ]. Asian men had a significantly higher 15-year survival rate compared to White men. Māori and Pacific men had higher rates of high grade PCa, which was associated with worse survival. A multivariable analysis adjusting for age, socio-economic deprivation, and cancer grade found ethnicity was independently associated with PCa-specific survival [ ].
3.3
Genetic Risk: Pathogenic Variants (PV)
Ten studies evaluated the association between pathogenic germline variants and PCa [ , ]. They included one systematic review with meta-analysis [ ] limited by an overall moderate risk of bias, seven cohort studies carrying an overall moderate [ , ] or critical risk of bias [ ] (summarized in Figure S1 ), and two modeling studies limited by an overall high risk of bias [ , ] (summarized in Figure S2 ).
3.3.1
HOXB13 Mutations
HOXB13 mutations have been associated with PCa. One study reported a 3-6-fold increased risk of PCa among HOXB13 G84E mutation-carriers and increased risk for mutation-carriers with FH of PCa [ ]. The authors reported a 62% absolute risk of PCa by age 85 in HOXB13 G84E -carriers and an absolute risk of 98% for those with two relatives diagnosed with PCa at a young age, compared to 15% average risk for non-carriers. Nyberg et al. (2023) reported a 39% risk of PCa in HOXB13 G84E -carriers vs. 16% in noncarriers [ ]. Another group reported increased odds of PCa and greater risk for more aggressive and advanced disease in patients carrying a rare African ancestry-specific HOXB13 variant [ ] ( Table S4 ).
3.3.2
DNA Damage Repair (DDR) Mutations
DDR mutations include mismatch repair (MMR), Homologous Recombination Repair (HRR), and checkpoint factor pathways, amongst others. Alteration in these pathways have been associated with PCa. Plym et al. (2022) found DDR gene mutations was more likely to be present in lethal vs. indolent PCa cases (18.5% vs. 9.6%, p=0.00043) [ ]. Additionally, HRR (p=0.003), Fanconi anemia (p=0.002), and checkpoint factor (p=0.02) pathway mutations were significantly associated with lethal PCa. HRR and Fanconi anemia pathways (both associated with BRCA2 mutations) were more prevalent in lethal PCa among European ancestry, MMR pathways more prevalent in lethal PCa among African ancestry, while checkpoint pathways (namely ATM ) were associated with lethality in both groups [ ]. Giri et al. (2022) reported nearly two-fold differences in PVs between White and African-American men. The authors also found differences in affected genes by race, with BRCA1/2, PALB2 , and ATM mutations in African-American men and BRCA2, ATM, HOXB13, CHEK2, TP53 , and NBN in White men [ ]. African-American men had 1.5-times the rate of carrying variants of unknown significance (VUS) and a ten-fold greater rate of multiple VUS compared to White men [ ]. DDR mutations were associated with younger age at diagnosis [ ].
3.3.2.1
Homologous Recombination Repair (HRR; BRCA1/2 )
BRCA1/2 mutation-carriers tend to be diagnosed with PCa at an earlier age [ ]. Nyberg et al. (2023) reported a 7-fold and 4-fold increased risk of PCa in BRCA2 -carriers compared to non-carriers, when under age 65 or ≥65, respectively [ ]. BRCA2 mutations are more common in patients of European ancestry than in those of African ancestry (5.1% vs. 0.9%) [ ]. Several studies have therefore investigated PCa screening strategies for BRCA1/2- positive men. Bancroft et al. (2014) found a positive predictive value (PPV) of 48% for prostate biopsy with a PSA ≥3 in BRCA1/2 -carriers. Men diagnosed with PCa at age <50 were all BRCA1/2 -carriers [ ]. When stratifying by age, one group found screening with mpMRI offered the best clinical utility for BRCA1/2 -carriers <55 years of age, given their PSA tends to be low; for patients ≥55 years old, PSA screening prior to mpMRI was optimal. Of note, 23% of BRCA1 -carriers were ≥GG2 while 71% of BRCA2 -carriers were ≥GG2 [ ].
3.3.2.2
Mismatch Repair (MMR) Mutations
Three MMR genes have been associated with PCa, including MSH2, MLH1, and MSH6 . Among MMR-mutation carriers with PCa, MSH2 is the most common followed by MLH1 and MSH6 , with all conferring increased risk of developing PCa, respectively [ ] ( Table S4 ). There were no differences in mean age at diagnosis, GS ≥8 disease, perineural invasion, or extracapsular extension between MMR mutation-carriers and non-carriers. While there was no significant difference in GS ≥8 disease between MSH2, MLH1, and MSH6 mutations (p=0.07), all high-grade PCa were in MSH2 -carriers [ ].
3.4
Polygenic Risk Score (PRS)
Thirteen observational cohort studies [ , , , ] and eleven modeling studies [ , , ] examined the role of PRS in predicting PCa. Of the total 13 observational studies, one [ ] carried an overall moderate risk of bias, six [ , , , , , ] were at serious risk, and six [ , , , ] were at critical risk of bias (summarized in Figures S1-2 ). While one modeling study [ ] carried low risk of bias, the remaining ten [ , , , , ] were at high risk of bias (summarized in Figure S2).
3.4.1
PRS + PSA
3.4.1.1
PCa Incidence
Several studies have examined the capacity of PRS to facilitate PCa and csPCa detection. Ahmed et al. (2020) reported that higher PRS predicted increased risk of PCa, particularly in the upper PRS quartile [ ]. PCa cases had higher PRS than controls and patients with a positive FH of PCa tended to have higher PRS [ ]. Nordström et al. (2014) found that among patients with PSA 1-3 ng/mL, PRS was predictive of PCa, independent of age, prostate volume, % free PSA, and FH [ ]. Patients in the top 10% of PRS had 4.1-fold greater odds of PCa compared to the 40-60th percentile. However, PRS was unable to discriminate between csPCa and non-csPCa [ ]. Several other studies reported no relationship between PRS and GS or stage or aggressiveness [ , , ]. However, PRS was more predictive of PCa than PSA alone (AUC = 0.630, (95% CI: 0.600-0.660) vs. AUC = 0.575, (95% CI 0.537-0.612)) and a model including PSA density and PRS further increased predictive power (AUC = 0.747, (95% CI: 0.713-0.777)) [ ]. In a Korean cohort, Song et al. (2022) found that a PRS >95th percentile conferred a 4.92-fold greater risk of csPCa versus the rest of the population [ ].
PRS may also be used to reduce PCa overdiagnosis. Numerous studies reported lower rates of overdiagnosis in higher PRS categories compared to lower PRS [ , , ]. Callender et al. (2019) demonstrated cost-effectiveness of PRS-driven PCa screening which could reduce overdiagnosis by nearly 60% [ ]. Interestingly, Chou et al. (2022) reported PSA and PRS were equally effective at predicting PCa 10+ years from time of PSA or PRS-based screening, but PRS was more effective at predicting risk earlier in life, though not PCa aggressiveness [ ].
3.4.1.2
PCa Mortality
Klein et al. (2022) postulated that while high-risk PRS significantly increases odds of PCa detection compared to average-risk PRS ( Table S5 ), PRS is not strongly associated with lethal PCa [ ]. They reported that PSA is a better predictor of PCa metastasis and lethality than PRS (AUC 0.78 vs. 0.63). Combining PRS and PSA did not improve PCa mortality prediction [ ]. In an analysis of the SELECT and PCPT clinical trials, PRS only predicted outcomes in the SELECT trial for men in the highest risk quartile [ ].
Ito et al. (2023) created a predictive PRS model based on 6.7 million SNPs from multi-ancestral studies, with a focus on those involving AR-binding sites. Patients in the top PRS decile had significantly greater risk of future PCa-related mortality in cancer-free patients [ ]. Ma et al. (2023) investigated a model based on PRS + baseline PSA to predict lethal PCa over a median follow-up of 33 years, which found an overall increased PCa risk (OR 1.91, 95% CI 1.49-2.44) and lethal PCa risk (OR 1.79, 95% CI 1.28-2.49) based on PRS [ ]. Interestingly, there was a stronger association between PRS and lethal PCa for men with baseline PSA <1 (OR 2.23, 95% CI 1.19-2.49) vs. men with baseline PSA ≥1 (OR 1.61, 95% CI 1.07-2.42). For non-lethal PCa, the OR was 1.26 (95% CI 0.76-2.08) for PSA <1. Overall, PRS was associated with both total and lethal PCa risk [ ].
3.4.2
Polygenic Hazard Score (PHS)
Karunamuni et al. (2021) utilized a modified polygenic hazard score (PHS) model and found significantly improved hazard ratios for PCa prediction, though the PPV for PSA testing with this model did not improve [ ]. Huynh-Le et al. (2022) compared different combinations of high vs. low PHS scores in four trials with the highest hazard ratios observed when comparing the top quintile to the bottom quintile [ ] ( Table S5 ). With this model, the trials with men of African and Asian ancestry had lower hazard ratios compared to European Ancestry. Furthermore, PPV of PSA was higher in the top 20% (PPV=0.19) and top 5% (PPV=0.26) of genetic risk compared to PSA alone (PPV=0.12) [ ].
3.4.3
PRS + FH
Some studies investigated PRS + FH models in PCa detection. One study found PRS became more predictive of PCa and high-grade PCa than positive FH when PRS was >1.4 [ ]. Positive FH alone was not useful for differentiating between high-grade and low-grade PCa risk. The authors found that a screening strategy targeting men with positive FH or PRS >1.4 was best at detecting the most PCa and those who would develop high-grade PCa. Importantly, patients with PRS <0.6 and negative FH had lower risk of PCa and a lower need for PCa screening [ ]. Huynh-Le et al. (2022) found that adding FH to their PHS yielded better PCa prediction than FH alone across all four trials studied [ ].
3.4.4
PRS/PHS + Race/Ethnicity
Several studies evaluated PRS/PHS and Race/Ethnicity for PCa prediction. A multi-study PRS model found PRS was highest for men of African ancestry, followed by American, South Asian, European, and East Asian ancestry [ ]. There was a high correlation between PRS and PCa incidence ( R 2 =0.900) and PCa-related mortality ( R 2 = 0.946) [ ]. Amrousy et al. (2022) found two novel SNPs in an Egyptian population to be independent PCa risk factors, but not for PCa recurrence [ ]. Similarly, Karunamuni et al. (2022) identified three novel African ancestry-specific SNPs which they added to a 46-SNP European ancestry-based PHS model to create the PHS-46+African model, which improved PCa prediction in African-ancestry populations, though this model was approximately 7-times better at risk stratification in some patients of African ancestry compared to others [ ]. Kim et al. (2022) compared PRS predictive power based on ancestry and found improved PCa risk-prediction with multi-ancestry or ancestry-matched PRS models [ ].
Pagadala et al. (2023) created a PHS model based on 290 SNPs (PHS290) [ ]. Average PHS290 was lower among Asian men and higher among Black men. PHS290 was an independent predictor of PCa, mPCa, and lethal PCa regardless of race/ethnicity and FH. PCa risk was significantly higher for men with higher PHS across all racial and ethnic subgroups [ ], as shown in Table S5. A European ancestry-based model found that PRS-calculated relative risk of PCa predictability decreases with increasing age, but this finding was only statistically significant among men of European ancestry [ ].
3.4.5
PRS + Multiple Clinical Factors
To provide better risk stratification and predictive accuracy, investigators have studied the role of adding PRS to several clinical risk factors. One model utilized PRS and 10 clinical risk factors, which performed significantly better (AUC: 0.904, 95%CI: 0.887–0.921) than other models without PRS [ ]. This model predicted up to 5-fold increased odds of PCa detection for patients in the top PRS quintile [ ] ( Table S5 ). Akamatsu et al. (2022) found that their best model for predicting PCa included PSA density, PRS, digital rectal exam, and age with an AUC of 0.784, (95% CI: 0.753-0.813), outperforming PSA alone or PRS and PSA [ ], as described in Section 3.4.1.1 . Dite et al. (2023) demonstrated efficacy in utilizing a PCa risk prediction model incorporating age, FH, and PRS, which identified a 2.7-fold increased PCa risk in the top decile compared to a population average [ ] ( Table S5 ). Furthermore, only men in the top three PRS deciles had greater risk than the population average and men in the lowest three PRS deciles had under half the risk of the average population [ ]. Finally, Nyberg et al. (2023) created 5 and 10-year PCa predictive models based on varying combinations of clinical factors. The best-performing models included age, FH, PV, and PRS, though models with age, FH, PRS, and no PV performed almost nearly as well [ ] ( Table S5 ).
4
Discussion
4.1
Family History
A positive PCa FH is a well-established risk factor for developing PCa. In this systematic review, we found evidence that a positive FH increases odds of csPCa diagnosis by 1.3-8.4-fold [ ]. This risk increases further with ≥2 first-degree relatives with PCa [ , ]. Patients with second-degree FH of PCa also have increased risk of PCa, though this risk is lower than in first-degree relatives [ ]. Interestingly, Castro et al. (2016) found 52% of FH-positive patients diagnosed with PCa had PSA level <3.0 ng/mL and otherwise would not have undergone a prostate biopsy, thus arguing that prostate biopsy is a feasible screening tool for patients with FH of PCa regardless of PSA [ ]. The four selected FH studies were limited by FH serving as a secondary outcome, patient reporting bias, and lack of granularity regarding GG, pathology, and age at diagnosis of PCa-positive family members.
While family history may be viewed as a proxy for genetic factors, in many cases it also comprises shared environmental factors, such as diet, stress, socioeconomic status (SES), neighborhood deprivation, and resource access amongst other factors [ ]. Documenting PCa FH is important when counseling patients on their risk—it is oftentimes more readily available and comes without the financial costs of genetic testing. However, in instances where FH is incomplete and/or unknown, genetic testing may close the gap.
4.2
Race/Ethnicity
The association between race/ethnicity and PCa has been extensively studied, demonstrating higher rates of PCa incidence, aggressiveness, younger age at diagnosis, and worse outcomes in Black patients and lower risk of PCa in Asian patients [ , , , ]. While Giri et al. (2022) found no differences in GS between Black and White races, this could be explained by significantly greater rates of PCa FH in White men in this cohort [ ]. This has prompted PCa screening guideline adjustments and community efforts to increase PCa screening education and participation in racial and ethnic minority populations to eliminate related disparities [ , ].
Nevertheless, race is a social-construct and it’s important to recognize the limitations of race-based medicine. Race and ethnicity are limited proxies for genetic contribution to disease development as they lack nuance regarding true contributions of ancestral genetics, whether it is bias related to self-identification or misunderstanding or lack of knowledge regarding ancestry. Additionally, race and ethnicity act as proxies for socioeconomic, environmental, and structural contributions to disease development, especially in the United States where racial and ethnic minorities disproportionately experience lower SES and face both environmental and structural bias as it pertains to hazardous exposure, stressful environments, and worse access to healthcare, among other factors. On the other hand, race and ethnicity may be more useful in investigating how individuals interact with the healthcare system. For instance, PCa screening behaviors amongst racial and ethnic minorities may be influenced by differences in patient-provider cultural approaches as well as mistrust in the healthcare system based on its historical mistreatment of racial and ethnic minority populations [ ]. In one study, non-Hispanic Black and Hispanic patients were less likely to experience shared decision-making regarding PSA screening and were therefore less likely to undergo PSA screening. Among patients who underwent shared decision-making, there were no racial disparities observed in PSA screening [ ]. While these studies did not fulfill our PICO criteria, it’s important to recognize these factors and how they influence our understanding of racial and ethnic disparities in PCa and PCa screening.
To eliminate or decrease confounding, some studies investigating racial/ethnic disparities in PCa adjusted for clinical factors. When adjusting for age, facility type, and diagnosis year, non-Hispanic Black, non-Hispanic other, and Hispanic-White, -Black, and -other patients had greater rates of Stage IV PCa at presentation compared to non-Hispanic White patients [ ]. When adjusting for SES factors, racial disparities in Stage IV PCa at presentation were reduced, though nearly all comparisons remained statistically significantly different, with the exception of Hispanic-Blacks. This suggests that both genetic and SES factors may contribute to advanced PCa presentation and racial/ethnic disparities. To highlight this, Hispanic-Black patient disparities in presentation of mPCa disappeared after adjusting for key SES factors [ ]. Furthermore, Chandra et al. (2021) reported racial differences in healthcare access and utility by demonstrating that Black patients across all insurance categories were less likely to receive mpMRI vs. commercially-insured White patients, which could help explain why Black patients tend to be diagnosed with PCa at more advanced stages because 12-core template prostate biopsies may miss mpMRI-detectable disease that could’ve been appropriately sampled [ ]. Even in some equal-access health care systems, which in theory may limit SES disparities, African-American men had increased risk of PCa detection and higher stage at presentation compared to Caucasian men [ , ]. However, these disparities were not noted in men aged 40-54 years, suggesting that early PSA testing in African-American men may allow earlier detection of PCa before the disparities noted in the older cohort arise [ ].
Evidence suggests that germline genetic testing is not equitable among races [ ]. Most studies do not engage in targeted recruitment to increase under-represented minorities in study populations, resulting in under-representation of non-White populations [ ]. Moreover, non-White populations are also under-represented in clinically-indicated genetic germline testing and in PCa clinical trials, which may further exacerbate racial and ethnic disparities [ , ].
To address racial and ethnic PCa disparities, some have studied implementation of culturally-sensitive community outreach programs to increase education of minority populations and encourage screening and patient engagement pertaining to PCa with encouraging results, though more studies are needed to determine if these programs yield earlier PCa detection and improved outcomes [ ].
4.3
Pathogenic Variants (PV)
Given the increased risk of PCa associated with positive PCa FH, germline genetic mutations have been suspected to contribute to heritability and disease development. Numerous studies have revealed associations between rare, high-penetrance pathogenic variants of genes involved in DNA damage repair and PCa, most notably BRCA1/2, HOXB13 , and MMR pathway mutations. These variants are quite rare—approximately 2% of PCa patients may carry at least one PV [ ]. Patients with PVs are more likely to present with earlier-onset disease, higher PSA, more aggressive cancer, and increased risk of metastasis [ ].
HOXB13 variants are associated with a 3-6-fold increased risk of PCa with further increased risk in patients with FH of PCa [ , , ]. Risk of PCa amongst HOXB13 -carriers is higher in more recent birth cohorts, suggesting contribution of differing environmental and/or lifestyle across generations [ ]. Although HOXB13 mutations are associated with increased PCa incidence, evidence varies regarding its association with PCa aggressiveness. For example, Akamatsu (2022) found no association between HOXB13 mutation and high-risk PCa, though the population was likely underpowered [ ]. Nyberg et al. (2019) found HOXB13 mutations did not predict more aggressive PCa compared to non-carriers [ ]. On the other hand, others have demonstrated HOXB13 mutation-carriers are more likely to have higher PSA at diagnosis, GS ≥7 disease, positive surgical margins, and overall more aggressive disease compared to non-carriers [ ]. Others have described how HOXB13 mutation variants differ among specific races and ethnicities [ ]. To this effect, a rare African-ancestry specific variant ( HOXB13 ×285K) was found to increase risk of PCa, more aggressive, and more advanced disease [ ]. These ancestry-specific differences may explain discrepancies in PCa outcomes among HOXB13 -carriers as certain mutations may have greater impact in the HOXB13 pathway.
Significantly more evidence exists for DDR mutation-carriers and increased risk of PCa incidence and lethality [ ]. Within the HRR pathway, evidence suggests BRCA2 -carriers have the greatest risk of PCa including greater risk than BRCA1 -carriers [ ]. PCa risk is greatest at younger ages for both BRCA1/2 -carriers. BRCA2- carriers are at greater risk of PCa at any given age while increased risk with BRCA1 -carriers is limited to younger ages [ ]. Mitra et al. (2017) reported a PPV of 47.6% when offering prostate biopsy to men with FH of BRCA1/2 mutations and PSA >3. Over a 33-month follow-up, 82% of detected PCa were mutation-carriers and 73% were csPCa [ ]. BRCA1/2- carriers may also benefit from additional testing with mpMRI and/or prostate biopsy given the increased risk for csPCa and mPCa even with low PSA, but further studies are needed to elucidate optimal screening regimens in this patient population.
BRCA mutations also impact PCa-related outcomes [ ]. BRCA2 PVs have greater association with aggressive tumor behavior and higher GS compared to BRCA1 -carriers [ , , ]. BRCA2 mutations are associated with increased risk of mPCa and worse overall survival [ , ]. Notably, however, Han et al. (2022) reported no differences in stage or grade group between BRCA2 -carriers and non-carriers, though the BRCA2 -mutation group may have been underpowered [ ]. Additionally, in advanced PCa, they also observed no differences in age at diagnosis between BRCA2 -carriers and non-carriers. BRCA2 -carriers with mPCa tended to have lower PSA than in non- BRCA2 carriers with mPCa, though this was not observed for localized PCa [ ]. BRCA1/2 mutations also confer worse outcomes in mCRPC with shorter PSA-progression-free survival and overall survival [ ].
Studies evaluating MMR mutations and PCa screening are limited. Nonetheless, MSH2, MLH1 , and MSH6 are most associated with increased PCa risk. Rosty et al. (2014) found significant differences in high-risk PCa features between MMR-carriers and non-carriers [ ]. Several studies have also associated MSH2 and MSH6 mutations with more aggressive PCa and worse outcomes [ ]. Others found lower levels of functional MLH1 in node-positive vs. node-negative PCa cases [ ]. These studies, however, were limited by small sample sizes due to their rarity. Interestingly, Wu et al. (2021) found that patients with an older age at diagnosis were more likely to have MMR-mutations than other DDR mutation pathways, such as HRR [ ].
It’s important to consider that many PVs have been investigated in racially and ethnically-homogenous groups—predominantly in those of European ancestry. Studies involving more diverse patient populations are revealing hundreds of VUS, many of which have not previously been published [ , ]. For example, one study reported the VUS rate in Black men was 1.5-times higher than the PV rate observed in White men [ ]. VUS may play a larger role in PCa development in men of African and other non-European ancestries as these populations have been historically under-studied compared to White men/European ancestry. Future studies of racial and ethnic minorities may reveal several VUS to be PVs in PCa development. There exists a crucial need to identify novel genes, VUS, expand gene panels, and increase diverse population representation to improve understanding of germline mutation contributions to PCa and provide genetic counseling most relevant to a patient’s ancestral composition.
Overall, despite PV rarity, its presence confers a high risk for PCa and worse PCa-related outcomes. Hence, patients with germline PVs may benefit from an earlier and/or more aggressive screening regimen. Future studies should investigate optimal PSA cutoffs and timing for mpMRI and biopsy indications.
4.4
Genetic/Polygenic Risk Score (PRS)
While high-penetrance PVs are rare and account for a small percentage of PCa inheritability, evidence suggests that a combination of numerous, common, low-penetrance SNPs may account for a majority of PCa risk, oftentimes independent of FH [ ]. PRS models combine the risks from individual SNPs into a single genetic risk score, which may be used to predict a patient’s risk or odds of PCa. SNPs are identified based on genome-wide association studies and selecting for common gene variants with the greatest association with PCa. PRS models have been proposed to improve risk stratification for patients undergoing PCa screening as well as predict risk of worse PCa outcomes. While genetic testing to determine PRS is relatively low cost, it has yet to be widely adopted into clinical practice, despite reports touting its cost-effectiveness in PCa detection and reduction in over-diagnosis [ , , ].
Implementation of PRS models into clinical practice may be hampered by heterogeneity. For example, we found PRS models varied from 29 to 6.7 million incorporated SNPs. PRS percentile cutoffs for reporting risk also varied widely between usage of quartiles, quintiles, or deciles. Of course, PCa risk increased as PRS percentile increased, but the ideal number of SNPs for a given PRS model and the ideal reporting of percentiles has not been established. Further, many PRS models were created with a primary endpoint of PCa detection [ , , , , , , ], though several investigators acknowledged PRS often failed to differentiate between higher GS, limiting its clinical utility [ , , , ]. However, Chen et al. (2023) reported a 1.23-fold increased risk of aggressive PCa in the top PRS decile compared to the 40-60th percentile, suggesting some patient comparisons yield risk stratification by PCa-aggressiveness [ ]. Studies were discordant regarding associations between PRS and PCa metastasis and mortality, with some reporting no or limited association [ , ] and others reporting increased risk of PCSM with higher PRS [ , ]. Further studies are needed to elucidate whether PRS is beneficial in predicting these crucial endpoints.
To improve predictive PRS models, investigators added non-modifiable risk factors such as FH, race/ethnicity, and other clinical factors to create hybrid risk models. Combined FH-positive and PRS models performed better than PRS alone and PRS in FH-negative models [ , ]. Low-PRS and FH-negative patients had low risk of csPCa, suggesting those with lower PRS-deciles could benefit from PCa screening de-intensification. Somewhat unsurprisingly, the best predictive models incorporated PRS and multiple clinical factors [ , , , ]. Importantly, such models may identify individuals at the extremes of high or low risk, suggesting possible benefits from screening escalation or de-escalation, respectively. For patients within the “gray-zone” PSA of 4-10 ng/ml, enhanced risk stratification may improve earlier csPCa detection while limiting overdiagnosis of non-csPCa.
There is evidence PRS may identify high-risk men and worse PCa outcomes prior to their development. One group observed a stronger association between PRS and lethal PCa for men with baseline PSA <1, suggesting that PRS can help identify higher-risk men even when initial PSA levels suggest low risk; this may help identify such men earlier and possibly prior to more aggressive PCa [ ]. Similarly, Chou et al. (2022) reported improved predictive utility of PRS for men at a younger age, as OR for PCa with higher PRS decreased as age increased [ ]. This suggests that PRSs may be more beneficial in risk stratification for younger men because if germline genetics play a predominant role, then patients are more likely to present with earlier-onset disease.
Most PRS models are based on genetic variants in populations of European-ancestry. Several studies have demonstrated that these models do not perform as well in more diverse populations and perform best in those of European-ancestry [ , , , , ]. Adding ancestry-specific SNPs to PRS models may improve their performance [ ]. Multi-ancestry PRS models perform better among more diverse populations [ , , ]. Finally, PRS models concordant with the study population perform best for a given specific ancestral population [ ]. These studies highlight the two-fold importance of diversifying genome-wide studies—first, to identify novel PCa-related SNPs and second, to improve PRS predictive utility in diverse populations and reduce racial and ethnic disparities.
5
Conclusions
Family history, race/ethnicity, germline pathogenic variants, and polygenic risk scores all offer significant insight regarding risk of PCa development and PCa outcomes. Implementing a prostate cancer early detection algorithm considering these factors may identify patients likely to benefit from an earlier and/or more aggressive screening regimen. Additionally, combining these risk factors into one predictive risk model may offer the best predictive utility compared to individual risk factors alone.
Funding
S.S.S. is supported in part by the Prostate Cancer Foundation, the National Institutes of Health ( R37CA283857 ), the University of Michigan Prostate S.P.O.R.E. ( P50 CA186786-05 ), the University of Michigan Comprehensive Cancer Center core grant ( 2-P30-CA-046592-24 ), the Department of Defense, Robert Wood Johnson Foundation as part of the Harold Amos Medical Faculty Development Program (AMFDP), the Urology Care Foundation Rising Stars in Urology Research Award Program and Astellas, Inc.
Disclosures
Artificial intelligence was not utilized during the preparation of this manuscript. S.S.S. is on a study advisory committee for Bayer Pharma and has a non-sponsored research agreement with GenomeDx. The other authors have declared that no conflict of interest exists.
CRediT authorship contribution statement
Nathan J. Graham: Writing – review & editing, Writing – original draft, Data curation, Conceptualization. Lesley H. Souter: Writing – review & editing, Writing – original draft, Supervision, Methodology, Formal analysis, Data curation, Conceptualization. Simpa S. Salami: Writing – review & editing, Writing – original draft, Supervision, Resources, Project administration, Methodology, Data curation, Conceptualization.
Declaration of competing interest
No Competing Interest to Declare.
Appendix
Supplementary materials
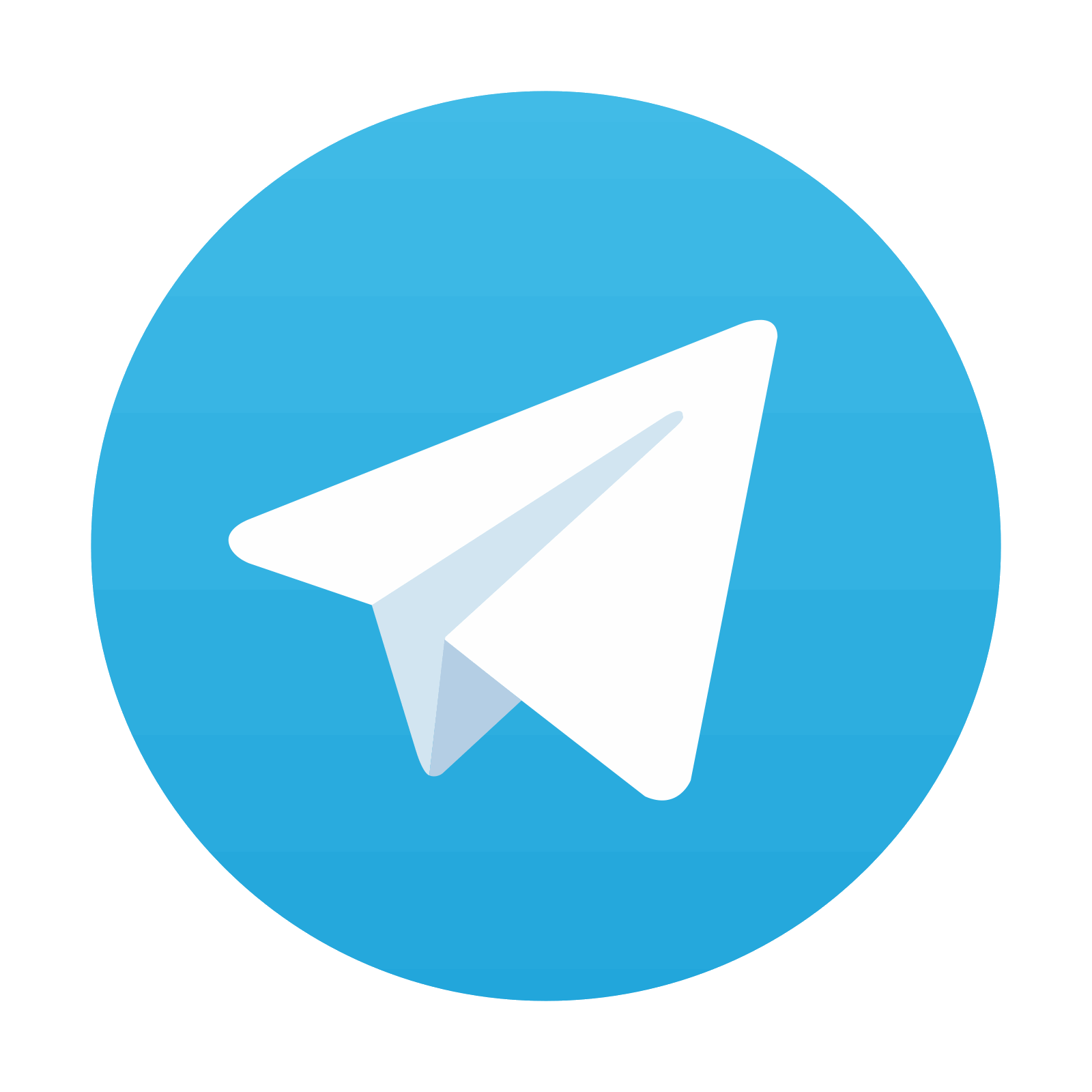
Stay updated, free articles. Join our Telegram channel
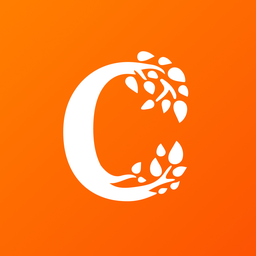
Full access? Get Clinical Tree
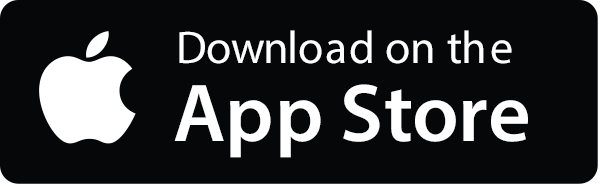
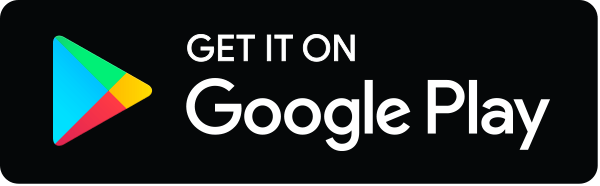
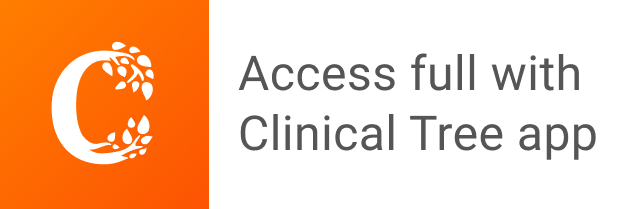